6G Antenna Requirements
Antenna dimensions for future communication are expected to be tiny, from μm to mm.31 This is attributed to the high operating frequencies (the higher the frequency, the smaller the wavelength). Thus, the first consideration is size. Fabricating and measuring a tiny antenna is a critical task that requires appropriate materials, technologies, facilities and equipment.
The next consideration is gain. 6G is expected to deliver extremely high spectral/spatial efficiency. To achieve this, a high gain and highly directional antenna is required. This mitigates high atmospheric absorption and high path loss.32 The orientation and location of devices connected in 6G are not fixed; antennas are, therefore, expected to be intelligently reconfigurable to provide full antenna beam coverage, high directivity and stable radiation patterns. Requirements for an antenna to perform optimally beyond 5G are summarized in Table III.
TABLE III - ANTENNA REQUIREMENTS FOR 6G AND BEYOND

Antenna Designs for 6G and Beyond
High path loss and atmospheric absorption are challenges identified for communication in the sub-THz and THz spectra.12, 33 In this article, antennas for 6G and future communication are divided into three types: patch, large arrays (emMIMO) and metamaterial.
Patch Antennas
The patch antenna is the most widely used. In 6G, it is designed for use at frequencies in the sub-THz and lower part of the THz bands respectively and requires the integration of several elements to improve its performance. A tooth-shaped inset-fed patch antenna, for example, is shown in Figure 5. It is fabricated based on a Rodgers 5880 substrate and operates at the sub-THz band with a resonant frequency of 0.19 THz.32 It has a return loss of 47.71 dB and a gain of 9.58 dB.
Another example is an ultra-wideband (UWB) coplanar waveguide-fed microstrip patch antenna, also fabricated on a Rodgers 5880 substrate and designed using ANSYS HFSS, which operates from 23 to 150 GHz (see Figure 6).34 Its gain increases gradually with frequency, peaking at 14.8 dB at 150 GHz with maximum efficiency at 90 GHz.
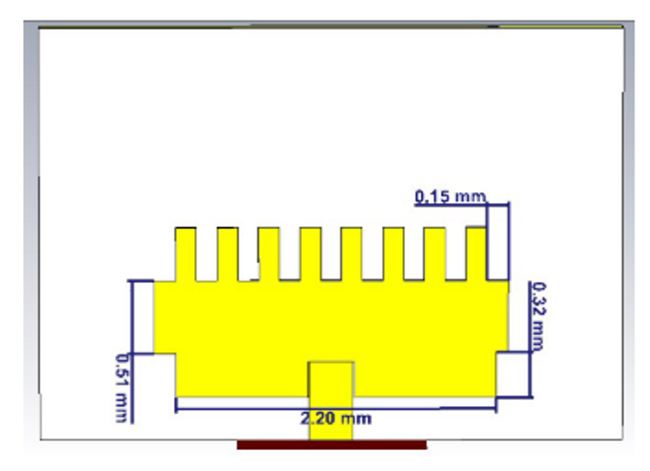
Figure 5 Tooth-shaped inset-fed patch antenna.32
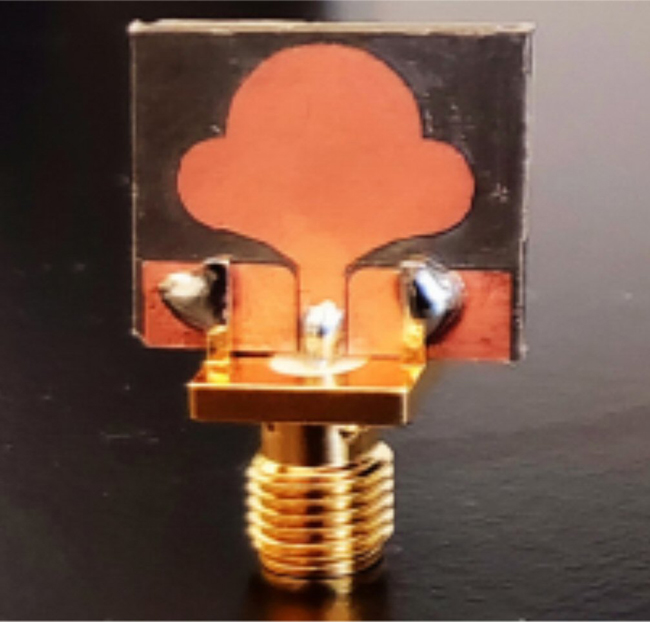
Figure 6 CPW-fed UWB microstrip patch antenna.34
The bandwidth of patch antennas is comparatively small.35, 36 However, Hedayatullah et al.37 introduced a wideband aperture-coupled patch antenna for the 6G sub-THz band. A peak gain of 7.95 dBi and |S11| ≤ -10 dB was recorded across a wide frequency range of 90 to 128.5 GHz. Also, described in the work of Jeyakumar et al.,38 a microstrip patch made of graphene, operating at 0.1 THz was designed and modeled using CST and ANSYS. A 27.7 dBi return loss, 98.30 percent radiation efficiency and 10.4 GHz bandwidth were achieved.
In related work,39 a reconfigurable circular patch antenna was developed for 6G cognitive radio applications (see Figure 7). With dimensions of 30 x 22 x 1.6 mm3 and a simple design, it allows for easy reconfiguration to other required frequencies by changing its square ring diameters with PIN diodes. The antenna operates from 3 to 11 GHz with a peak gain of 4.8 dBi.
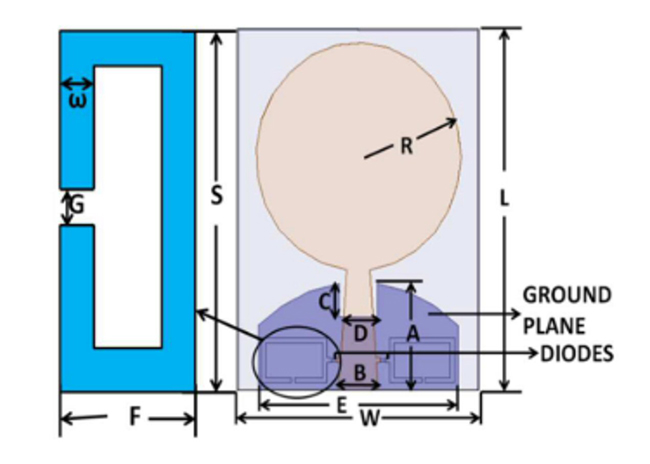
Figure 7 UWB frequency reconfigurable antenna.39
Large Arrays (emMIMO)
mMIMO is a cellular technology where the access points are equipped with a massive array of antennas33 to improve beamforming and coverage area. mMIMO technology is currently used in 5G networks; however, hundreds or even thousands of array antennas are envisioned for 6G to achieve ultra-high spectral resolution, wide coverage and highly directional signals.40, 41 This new technology is extremely massive MIMO (emMIMO). MIMO is a few hundred elements, mMIMO is several hundreds of elements, while emMIMO is a new term. It is expected that 6G emMIMO antennas will comprise several thousand array elements. This development is still in a preliminary stage.
The parameters used to determine the performance of emMIMO antennas include envelope correlation coefficient (ECC), which defines the correlation between adjacent antenna elements; diversity gain, which indicates the quality and reliability of the antenna; channel capacity loss (CCL), which defines the maximum limit of data that can be transmitted with almost zero loss in the communication channel and mean effective gain (MEG), which is the ratio of MIMO antenna power to isotropic received power of the antenna.30
The following are a few examples of research on the MIMO building blocks that may lead to effective mMIMO and emMIMO solutions:
Figure 8 is a tightly spaced 16-port 2 × 2 module array MIMO antenna on a 0.4 mm FR4 substrate.42 The antenna operates from 7.025 to 8.4 GHz. To achieve a high spectral efficiency of 56 bps/Hz, the module array is a 16 × 8 MIMO system consisting of 16 receive antenna and eight spatial streams.
In the work of Khaleel et al.,43 a double-port THz MIMO antenna made of graphene plasmonic on a 130 × 85 μm2 Teflon substrate is described. It has a low CCL of 0.006, a consistent radiation pattern, a compact size, a low envelope ECC of 0.000168, a high gain of 7.23 dB, and a wide impedance bandwidth of 0.6 THz.
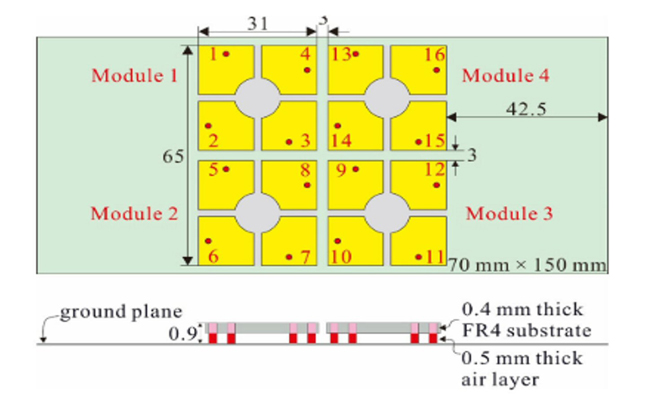
Figure 8 16-port 2 × 2 module array antenna.42
Wu and Zhang44 designed a 7.1 to 13 THz graphene-based antenna (see Figure 9) on a 10 μm thick SiO2 substrate measuring 100 by 100 μm2. A high gain of 8.3 dB was realized at 11 THz with a return loss of 30 dB. With an ECC of less than 0.005 dB and a diversity gain of 9.97 dB, the antenna is capable of functioning in mMIMO applications for THz point-to-point wireless communication.
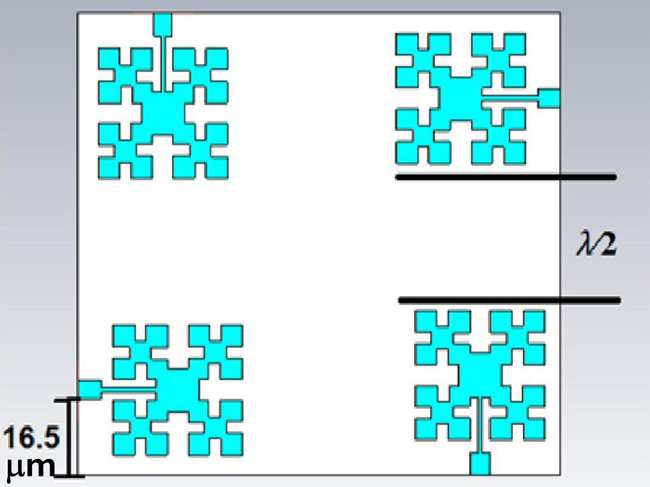
Figure 9 Graphene-based THz MIMO antenna.44
Metamaterial (MM) Antennas and Reconfigurable Intelligent Surfaces (RIS)
MMs are materials that have characteristics different from those found in nature and whose electromagnetic properties are described by their electric permittivities (ε) and magnetic permeabilities (μ).45, 46 MMs are capable of absorbing unwanted radiation and only permitting the desired radiation, improving antenna gain, controlling scattering, improving bandwidth and intelligently directing a signal to the desired user.47-49
Muqdad et al.50 described a novel metasurface reconfigurable antenna for 6G. Fabricated on an FR4 epoxy substrate, the metasurface concentrates the antenna’s main beam to enhance directivity. The antenna resonates at 1.35 GHz and does not require any mechanical movement; hence, it is intelligent.
Xie et al.51 proposed an ultra-wideband achromatic metalens antenna for operation between 50 and 102 GHz. The antenna consists of a convex-like metalens and a concave-like metalens integrated as a metalens group. A gain of 23.27 dBi and a return loss greater than 15 dB at 68 GHz were realized.
It was suggested by Muqdat et al.50 to use PIN photodiodes to create a metasurface antenna whose radiation properties could be optically controlled for 6G mobile communication applications (see Figure 10). The antenna operates from 0.978 to 1.73 GHz with a return loss greater than 10 dB, realizing a peak gain of 9 dBi at 1.35 GHz. The antenna is a rectangular patch incorporating a 3-order H-tree fractal slot. The radiator is positioned above a metasurface layer that has a small air gap between it and the radiator. The metasurface layer is a lattice structure formed from unit cells that are joined to each other by PIN photodiodes that enable antenna reconfiguration.
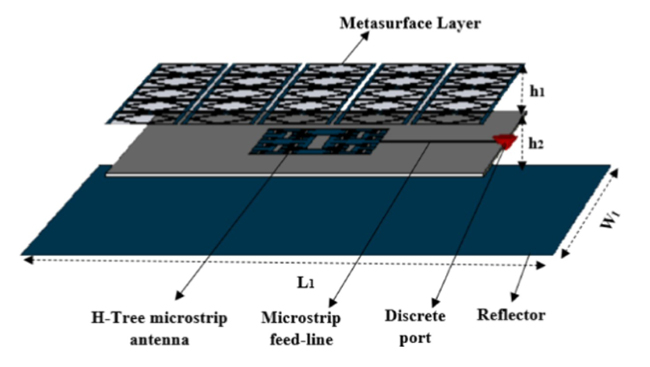
Figure 10 Photonic-controlled metasurface antenna for 6G.50
A high gain circularly polarized antenna with a μ negative MM slab and near-zero refractive index was proposed by Ghzaoui et al.48 To achieve high gain and circular polarization, the antenna used a substrate-integrated waveguide (SIW) approach loaded with a compact square split ring resonator combined with a hexagonal-shaped structure as a unit cell. The antenna was simulated over a wide frequency range of 100 to 280 GHz, recording a peak gain of 11.3 dBi at 223 GHz. Although high gain and good cross-polarization were achieved, the antenna was not reconfigurable.
To enhance the gain of many beams emitted from a linear feed antenna array while accounting for multi-layer transmission, a large-aperture metamaterial lens antenna (MLA) was developed by Lee and Kim.52 The antenna comprised 28 × 28-unit cells fed by a 1.6 dBi dipole antenna. The MLA delivered 14 dBi gain at a resonant frequency of 28 GHz. The high gain obtained was a result of a novel channel model they presented, which considered the radiation pattern, azimuth radiation pattern and meta feed.