Car manufacturers continuously explore and assess innovative technologies that could provide them with a competitive edge. Ultra-wideband (UWB) technology, a short-range wireless communication protocol, is one example. UWB already supports premium automotive features such as secure keyless car entry.
But UWB’s potential extends far beyond simple tasks. Thanks to its fine-ranging capabilities, the technology could also facilitate in-cabin radar sensing, making UWB an important enabler of in-cabin gesture recognition applications. Alternatively, its in-cabin radar sensing capabilities could be used to support child presence detection (CPD) solutions that issue an alert if an infant is left unattended in a parked vehicle.
Researchers and technology providers are actively investigating UWB requirements to accommodate these features. A team of researchers at imec recently announced the availability of a low-power UWB chip that uses a pulse-shaping mechanism to improve the technology’s ranging precision. Complementing this effort, they also demonstrated the added value of advanced signal processing algorithms for real-time, highly accurate car occupancy detection and breathing rate estimation.
ULTRA-WIDEBAND TECHNOLOGY: A POTENTIAL LIFESAVER
Drivers are regularly warned not to leave small children unattended in parked cars because of their heightened susceptibility to hyperthermia and heatstroke. If a car is exposed to the sun, in-cabin temperatures can reach a critical level in just 15 minutes. Leaving windows ajar does little to reduce this risk. Despite all awareness campaigns, nearly 1,000 children have died due to pediatric vehicular heatstroke since 1998 in the U.S. alone.1 These deaths could have been prevented with appropriate warning systems in place. The good news is that such warning mechanisms are on the brink of becoming a reality, as initiatives like the European New Car Assessment Program (Euro NCAP) spur car manufacturers to integrate CPD solutions as a standard feature.2
Early CPD systems mainly relied on ultrasound but these systems suffered from accuracy limitations caused by external vibrations and noise. Radar technology, particularly at 60 GHz, offers a viable alternative. It is less prone to external disturbances and provides accurate results. The disadvantage is that mmWave radar solutions are currently less suited for mass deployment due to their cost.
Cost is an area where UWB radar can provide benefits. UWB already has the advantage of being integrated into premium cars to support features such as keyless entry. This makes it a technology familiar to the automotive sector. Additionally, operating within the 6 to 10 GHz frequency range, UWB radar easily penetrates car seats. It is also inherently capable of detecting even the slightest movements, such as an infant’s chest rising and falling with each breath. Lastly, it comes at a lower cost than mmWave radar technology.
Technology vendors are already demonstrating and promoting the added value of UWB radar as a life-saving technology, even though practical implementations are not yet commercially available. Still, it is already clear that any viable solution will need to possess a transceiver architecture, allowing simultaneous transmission and reception. It should also prioritize extremely low power consumption to ensure seamless support for CPD systems running on electric car batteries. Achieving outstanding ranging precision will be a critical success factor as well.
POWER CONSUMPTION OF THE IR-UWB TRANSCEIVER

Figure 1 Architecture of imec’s IR-UWB 3Rx-1Tx transceiver. Source: imec.
Researchers at imec recently developed an IEEE 802.15.4z-compliant impulse radio (IR) UWB transceiver with low power consumption. The team believes that this is a key milestone to the realization of in-cabin UWB radar-on-chip applications. The IR-UWB transceiver uses a cost-efficient silicon implementation and operates over a frequency range of 6 to 9 GHz, consuming 8.7 mW/21 mW in continuous Tx/Rx mode.3 The imec researchers believe that is the lowest power consumption among state-of-the-art IEEE 802.15.4z radios. The block diagram of this transceiver is shown in Figure 1.
Fabricated in 28 nm CMOS technology and occupying a silicon area of 1.33 mm, the chip’s low power consumption results from an optimized, low-power and interference-resilient Rx architecture coupled with a digital polar transmitter architecture. A distributed, two-stage, all-digital phased locked loop (PLL) further reduces the chip’s power consumption and contributes to a reduced measurement time for localization.
PULSE-SHAPING ENHANCES UWB’S RANGING PRECISION
The availability of a low-power solution will be essential to making CPD and other in-cabin sensing solutions a reality, but equally important is the technology’s ranging precision. To support object localization and target ranging, UWB positioning systems rely on the time of flight (ToF) of RF pulses. Following a pulse’s transmission at a channel center frequency of around 6 GHz, a receiver filters and measures the signal’s time of arrival (ToA) to estimate the range distance between the transmitter (TX) and the receiver (RX).

Figure 2 Block diagram of a UWB transmitter and receiver. Source: imec.
To make this technique work inside a vehicle will be challenging. The in-car environment is prone to signal distortions caused by reflections from the floor, roof, windows and seats. These reflections result in multipath signals, making it more difficult to accurately measure the direct path signal and reliably estimate the target’s location and distance. Figure 2 shows a block diagram of an UWB TX and RX measuring the ToA of an RF pulse in a cluttered environment characterized by multipath signals. A multipath environment not only features a direct path line of sight (LOS) signal that should be measured to obtain a target’s distance and location; it also comes with non-line of sight (NLOS) signal components, reflecting off surfaces and scattering from objects. These multipath components make it more difficult to measure the direct path and reliably estimate an object’s distance and location.
To offset the impact of multipath components, pulse-shaping strategies are used to make the pulse as short as possible. The rapid change in amplitude improves the precision with which the signal’s ToA can be measured. The short duration of the pulse helps in measuring the direct path LOS well before the NLOS multipath signals arrive. The design of a pulse shape comes with quite some flexibility: as long as UWB standards and specifications are met, the shape of the pulse, as well as the design of the TX and RX circuitry, are largely left to the system and product developers to optimize.
An important element to consider is that the UWB’s frequency range has been divided into channels to manage interference between users. The TX must meet a spectrum mask, limiting the bandwidth and constraining the signal and ToA measurement. The RX also has a channel filter with limited bandwidth to remove potential interference in neighboring channels. In other words, the benefit of short pulse duration should be balanced against the occupied spectrum. As the pulse gets shorter, the spectrum gets wider and pulse-shaping mechanisms must account for this important limitation.
A PULSE-SHAPING APPROACH USING AN ANTI-PHASE PRECURSOR
To accurately estimate the arrival time of a pulse, it is important to consider the leading edge of the pulse. This is especially true in indoor and in-cabin environments prone to multipath conditions where subsequent multipath components can potentially corrupt the trailing edge and to some extent, even the peak of the pulse. To address this, imec researchers propose creating an asymmetric pulse shape with a steep leading edge to disentangle the multipath components from the main signal. They propose doing this with an anti-phase precursor pulse with the opposite carrier phase.4 This asymmetric pulse sharpens the pulse rise time, improves ToA measurement precision and decreases the interference from close multipath components while staying comfortably within the spectral mask for channelized signal compliance.

Figure 3 Hamming pulse with a precursor. Source: imec.
As a starting point, imec researchers used a pulse shape based on a Hamming waveform. The spectrum of this waveform has a good margin to the UWB spectrum mask requirement. The transmitted pulse shape consists of a main pulse and an auxiliary anti-phase pulse that is transmitted two nsec before the main pulse. This creates a short, negative-going precursor pulse that depresses the start of the main pulse and increases the slope of the rising edge up to the peak, all within the constraints of the spectral mask. The result is shown in Figure 3.
GENERATING A PULSE WITH AN ANTI-PHASE PRECURSOR
To generate this anti-phase precursor for the pulse, imec researchers built a circuit based on their wideband transceiver implementation. The TX and RX are supplied by a locked frequency source using a cascaded PLL topology with a crystal oscillator to generate the 499.2 MHz system clock and 6 to 9 GHz local oscillator (LO) frequency. Second-stage LO PLLs generate the LO locally for the TX and the RX separately, reducing power consumption and simplifying the clock distribution.
The biggest challenges in the UWB TX design are the trade-offs between spectrum and low-power performance and dealing with inter-pulse interference (IPI). The IEEE 802.15.4a/z standards define the channel bandwidth and a chip/pulse rate of 499.2 MHz. This requires the pulse duration to be approximately 4 ns, exceeding the chip period. Unlike single pulse patterns in the preamble, IPI can be encountered in the payload data stream due to consecutive pulses at the chip rate.

Figure 4 TX circuit design to generate a pulse with an anti-phase precursor. Source: imec.
imec’s UWB TX design is shown in Figure 4. It features a digital deserialization-serialization (DesSer) circuit to reduce the IPI. A two-bit amplitude and phase code at 499.2 MHz is first deserialized into two interleaved parallel paths running at 249.6 MHz. Each path employs the asynchronous pulse-shaper, producing 16 delayed sub-pulses, which together form a quantized Hamming pulse shape. The asynchronous shaping completely removes spectral images from which synchronous pulse-shaping systems suffer at the cost of a higher quantization noise floor. However, the quantization noise floor can be reduced by selecting sufficient quantization levels. The outputs of the two interleaved parallel asynchronous pulse-shapers are serialized by a digital serializer, either summing or subtracting them according to the polarity given by the phase code. Furthermore, the digital serializer extracts a new phase code signal, synchronizing it with the zero-crossing detection circuit, facilitating the phase transition without introducing spurious emissions.
An additional parallel path generates a smaller precursor pulse with opposite polarity. A similar DesSer circuit digitally combines the precursor and main pulse path before the power amplifier. Switching between the anti-phase precursor and main pulse is also synchronized by a zero-crossing detection circuit to avoid generating spurious emissions.
REALIZING AN IMPORTANT RANGING PRECISION IMPROVEMENT

Figure 5 Transmitter output signal and spectrum with and without precursor. Source: imec.
Experiments using imec’s IC implementation show that it effectively improves ToA measurement performance and UWB ranging precision. Figure 5 shows the performance improvement achieved by the TX while maintaining spectral compliance. The pulse mixing circuit, together with the resynchronization, maintains the sidelobe suppression of -35 dBc with and without precursor pulses. The addition of the precursor pulses reduces the rise time of the leading edge of the main pulse from 700 to 550 psec.
Using the precursor pulses has been shown to improve the precision of the difference in the ToA estimation by a factor of almost four. Care should be taken in extrapolating this difference result to general ranging performance due to limitations in measuring absolute ToA. However, the improvement is undoubtedly useful and arises from a combination of interrelated factors, including the pulse shape and the RX processing.
AN IEEE 802.15.4Z-COMPLIANT IR-UWB RADAR SYSTEM FOR IN-CABIN MONITORING
Building on this UWB transceiver design, imec researchers have developed an experimental IR-UWB radar system for real-time, in-cabin sensing.5 The system operates at a bandwidth of 499.2 MHz to comply with the IEEE 802.15.4z standard. It provides occupancy detection and breathing rate estimation for individuals occupying the driver and/or front passenger seats, along with passenger gesture detection, all compelling automotive use cases.

Figure 6 In-cabin demo setup. Source: imec.
The demo setup, simulating an in-cabin environment with a full-duplex, single-input, single-output (SISO) mono-static IR-UWB radar is depicted in Figure 6. The antennas are strategically placed on the sides, tilted toward the ground and inclined more toward the driver’s seat to include both seats in the main lobe of the antennas. The passenger and driver seats maintain a 45 cm edge-to-edge distance with the antennas positioned at 1.17 and 1.70 m from the center of the passenger and driver seats, respectively. Each TX and RX antenna is connected via 100 cm cables to the IR-UWB platform, which captures channel impulse responses (CIRs) every 10 msec, utilizing IEEE 802.15.4z-compliant SP0 packets. Following de-spreading and CIR accumulation, a captured CIR over fast time is estimated with a resolution of Tf = 1 nsec.
A crucial enabler to detect two closely located targets for a system with 499.2 MHz of bandwidth is advanced signal processing algorithms. This is especially true for an in-cabin environment where the driver and the passenger are in close proximity. With custom-built algorithms, imec researchers successfully achieved precise detection of both individuals, achieving a false alarm probability of more than 95 percent.
The researchers conducted two sets of experiments to evaluate the system’s capability in estimating breathing rates. In the first scenario, their objective was to validate the system’s accuracy in estimating breathing rates within a margin of less than 1 bpm. Employing a reference device with belt sensors to measure the subject’s breathing rate, the reference device recorded a breathing rate of 11.96 bpm, while the demo setup estimated it at 11.71 bpm, confirming its accuracy within the desired threshold.
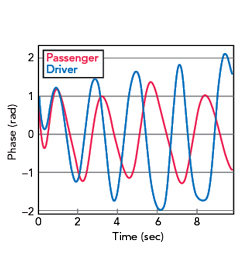
Figure 7 Passenger and driver breathing signals. Source: imec.
The researchers also successfully demonstrated the system’s ability to estimate the passenger and driver’s breathing rates simultaneously. This result is illustrated in Figure 7, which shows the extracted breathing signals for both individuals. The researchers did observe that body movements and interference between targets can distort the measurements, which has them considering the development of specific algorithms to mitigate these factors in future work.
Algorithms also played a crucial role in the in-cabin detection of a predefined gesture. The experiments conducted by the imec team revealed that their classifier achieved 99.9 percent accuracy in correctly detecting false gestures and 90.5 percent for the reference gesture. According to imec researchers, these numbers can be further improved with additional data collection. For future work, they plan to gather more data and define more gestures to enhance the range of possible interactions between the human-vehicle interface.
While there are possible improvements, the imec experiments demonstrated the effectiveness of the proposed IR-UWB radar system. The results showcase the potential of using a UWB-based radar system to enhance automotive safety and comfort, particularly in CPD applications. Efforts like this one from imec will form the basis for improvements in vehicle in-cabin safety.
imec will present results on its ultra-wideband technology at the 2024 IEEE International Solid-State Circuits Conference.6
References
- Heatstroke Deaths of Children in Vehicles, September 2023, Web: noheatstroke.org.
- “Test and Assessment Protocol – Child Presence Detection,” Euro NCAP, V. 1.1, November 2022, Web: cdn.euroncap.com/media/75474/euro-ncap-cpd-test-and-assessment-protocol-v11.pdf.
- M. Song, et al., “An 8.7 mW/TX, 21 mW/RX 6-to-9GHz IEEE 802.15.4a/4z Compliant IR-UWB Transceiver with Pulse Pre-Emphasis Achieving 1.4mm Ranging Precision,” IEEE Symposium on VLSI Technology and Circuits, June 2023.
- C. Marshall, E. Allebes, A. Sheikh, M. Song, M. E. Soussi and Nick Winkel, “A UWB Pulse with Precursor for ToA Measurement,” International Conference on Indoor Positioning and Indoor Navigation, September 2023.
- A. Farsaei, B. Meyer, A. Sheikh, M. E. Soussi, P. Zhang, G. K. Ramachandra, J. Govers and M. Hijdra, “An IEEE 802.15.4z Compliant IR-UWB Radar System for In-Cabin Monitoring,” IEEE International Symposium on Personal, Indoor and Mobile Radio Communications, September 2023.
- 2024 IEEE International Solid-State Circuits Conference (ISSCC), Web: https://www.imec-int.com/en/5G-and-wireless-iot-communication/ultra-wide-band-uwb-technology.