5G New Radio (NR) is comparable to the mobile communications industry using LTE to describe 4G technology or Universal Mobile Telecommunications Service (UMTS) to describe 3G. As a start, the release 15 specifications for 5G NR were approved in June 2018. These will continue to evolve to cover the detailed technical functionality of Standalone (SA) access for 5G NR devices.
Here are five key technical aspects of the 5G physical layer that enable this global communications standard to deliver an abundance of reliable, data rich and highly connected applications.
1. 5G NR WAVEFORMS
CP-OFDM: Downlink and Uplink
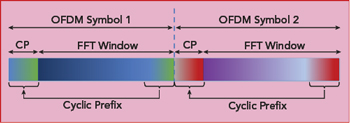
Figure 1 A CP-OFDM symbol contains a cyclic prefix on each side of the data.

Figure 2 Time and frequency comparison of OFDM (a) and DFT-S-OFDM (b).

Figure 3 NR bands above 6 GHz.
Researchers have been investigating different multicarrier waveforms in recent years, proposing many for 5G radio access. Waveforms that use orthogonal frequency division multiplexing (OFDM) work well for time division duplex operation. They support delay-sensitive applications and have demonstrated successful commercial implementation with efficient processing of ever-larger bandwidth signals. Also, the high spectral efficiency and MIMO compatibility of OFDM signals help meet the extreme data rate and density coverage needs of this new global cellular communications standard.
Thanks to channel estimation and equalization techniques, OFDM waveforms demonstrate great resiliency in frequency-selective channels. By attaching a copy of the end of the OFDM symbol to the beginning of the symbol (a cyclic prefix), a receiver can better tolerate synchronization errors and prevent intersymbol interference (see Figure 1). So the 3GPP settled on using the cyclic prefix OFDM (CP-OFDM) as the waveform for 5G downlink and uplink for modulation schemes up to 256-QAM.
DFT-S-OFDM: Higher Efficiency Uplink
OFDM waveforms suffer from high peak-to-average power ratios (PAPR). Because the RF power amplifier consumes the most power in a mobile device, system designers wanted a waveform supporting high efficiency amplifier operation while meeting the spectral demands of 5G. For uplink (i.e., user to base station), NR offers user equipment (UE) the option of using CP-OFDM or a hybrid format waveform called discrete Fourier transform spread OFDM (DFT-S-OFDM). Using DFT-S-OFDM, the transmitter modulates all subcarriers with the same data (see Figure 2). It lowers the peak-to-average ratio while retaining the multipath interference resilience and flexible subcarrier frequency allocation OFDM provides. Where the PAPR with CP-OFDM may be 11 to 13 dB, with DFT-S-OFDM it is only 6 to 9 dB.
2. FLEXIBLE SUBCARRIER SPACING, FRAME STRUCTURE
Operation in multiple frequency bands is a new aspect of 5G NR, from the existing cellular bands below 3 GHz, to wider bands between 3 and 5 GHz, to the mmWave spectrum. Figure 3 shows the current bands defined for NR operation above 6 GHz.
As the carrier frequency increases, so does system phase noise. For example, the difference in phase noise between carriers at 1 and 28 GHz is about 20 dB. This increase makes it difficult for a mmWave receiver to demodulate an OFDM waveform with the narrow, fixed subcarrier spacing (SCS) and symbol duration of LTE. Also, with moving users, the channel coherence time decreases as the carrier frequency increases because of the Doppler shift, meaning the system has less time to measure the channel and finish a single slot transmission at higher carrier frequencies. Using a narrow subcarrier spacing at mmWave results in unacceptably high error vector magnitude, with considerable performance degradation.
To address these challenges, the 3GPP standardized on a flexible subcarrier spacing that scales the space between orthogonal subcarriers, starting with the 15 kHz subcarrier spacing used for LTE and going to 30, 60 or 120 kHz spacing at mmWave. Leveraging the LTE numerology ensures NR deployments will coexist and be time-aligned with LTE networks.
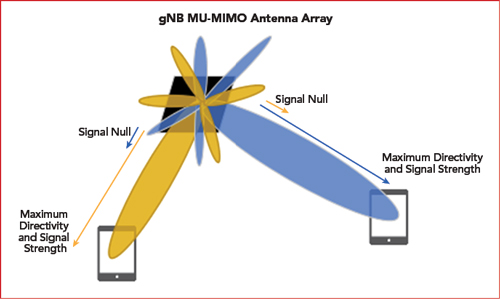
Figure 4 Spatial multiplexing using MU-MIMO.
3. MIMO
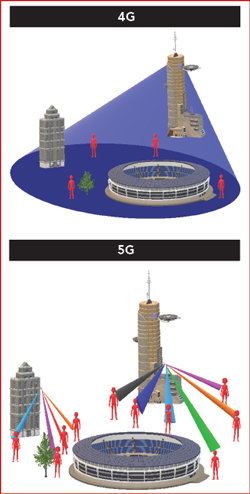
Figure 5 Spatial multiplexing with mMIMO increases gNB capacity.
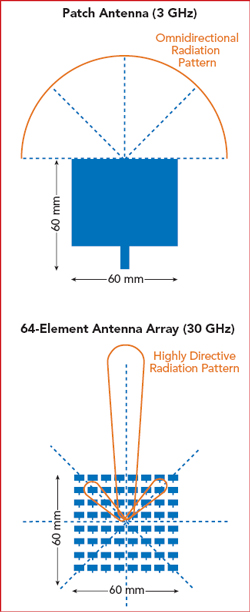
Figure 6 64-element array at 30 GHz has the same size aperture as a single 3 GHz patch antenna.
To increase capacity and spectrum efficiency, 5G NR uses the distributed and uncorrelated spatial locations of multiple users. Using multiuser MIMO (MU-MIMO) technology, the base station (gNB) simultaneously sends data streams to different users, maximizing the signal strength at each user’s location while reducing the signal strength (creating nulls) in the directions of the other receivers. This enables the gNB to talk with multiple UEs independently and simultaneously (see Figure 4).
mMIMO for 5G
Massive MIMO (mMIMO) refers to a communications scenario with many more gNB antennas than users. A large difference between gNB antennas and UEs can yield huge gains in spectral efficiency, enabling the communications system to simultaneously serve many more devices within the same frequency band than today’s 4G systems (see Figure 5). Industry leaders have demonstrated the viability of mMIMO systems for 5G using software defined radio and flexible software, which enable rapid wireless system prototyping.1
4. mmWAVE FOR 5G
5G systems operating at 28 GHz or other mmWave bands have the advantage of more available spectrum, enabling larger channels. While the mmWave bands have less spectral crowding than the bands below 6 GHz, communications systems using at these frequencies must contend with very different propagation effects: higher free-space path loss and atmospheric attenuation, weak indoor penetration and poor diffraction around objects. To overcome these undesired effects, mmWave antenna arrays focus their beams and take advantage of antenna array gain. Fortunately, the size of these arrays decreases as the frequency increases, enabling a mmWave antenna array with many elements to be roughly the same size as a single, sub-6 GHz element (see Figure 6).
As noted, the channel coherence time decreases significantly at mmWave frequencies, placing tough restrictions on UE mobility applications. As researchers continue to investigate new ways to improve mobility at mmWave, the first 5G mmWave deployments will likely serve fixed wireless access applications such as home broadband, backhaul and sidelink.
5. BANDWIDTH PARTS
As 5G applications grow, the diversity of devices and equipment will have to operate successfully across many different bands with varying spectrum availability. One example is the situation where a UE with limited RF bandwidth operates beside a more powerful device that can fill a whole channel using carrier aggregation and a third device that can cover the whole channel with a single RF chain.2
While wide bandwidth enables higher data rates for users, it comes with a cost. If UEs do not need high data rates, using wider bandwidth than required is an inefficient use of the RF and baseband processing resources. 5G NR introduces the concept of bandwidth parts (BWP), where the network negotiates for a certain UE to occupy one wideband carrier, separately configuring other UEs with a subset of contiguous resource blocks. This allows a greater diversity of devices with varying capabilities to share the same wideband carrier. This flexible network operation adjusting to the differing RF capabilities of UEs does not exist with LTE.
SUMMARY
Thanks to higher bandwidth channels and multiple numerology options, NR systems will operate in both sub-6 GHz and mmWave bands, appropriately handling multipath delay spread, channel coherence time and phase noise. NR leverages the latest developments in mMIMO and beamforming to maximize spectral efficiency and provide better quality of service for a larger number of users. Although creating the next generation of 5G devices presents significant design and test challenges, a platform-based approach to design, prototype and test these new wireless technologies is key to 5G becoming a reality within the next decade.
References
- 1. G. Xu, T. Li et al., “Full Dimension MIMO (FD-MIMO): Demonstrating Commercial Feasibility,” IEEE Journal on Selected Areas in Communications, Vol. 35, No. 8, August 2017, ieeexplore.ieee.org/abstract/document/7938334/.
- 2. 3GPP Technical Specification Group Radio Access Network, “NR, Physical Layer Procedures for Control,” TS 38.213 V15.0.0, https://portal.3gpp.org/desktopmodules/Specifications/SpecificationDetails.aspx?specificationId=3215.