The first thing to consider is, why millimeter-waves? First, there are many advantages associated with increasing the operating frequency for a majority of already well developed applications, including the potential for increased available bandwidth, and the improved resolution and directivity which can be obtained for a given antenna aperture. This can mean smaller, lightweight systems offering increased transmission capacity in the case of communication systems or improved resolution for radar or imaging systems. In the latter case, millimeter and/or sub-mm systems are often mandated to achieve useful resolution for image recognition, or identify explosive materials by their spectroscopic signatures.

Additionally, there are unique features associated with the transmission properties of the atmosphere in the millimeter spectrum region, generally accepted to mean wavelengths between 1 and 10 mm or the band of frequencies covering 30 to 300 GHz. Figure 1 places the mm-band relative to other electromagnetic radiation and, as an example, Figure 2 shows the effective zenith atmospheric transmission in dB/km for typical conditions at sea level and a height of 4 km. The impact of the 60 and 120 GHz O2 lines and multiple resonances of the H2O molecule can be seen.
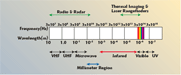
Such specific and unique resonances allow the remote sensing of atmospheric gases in the Earth and planetary atmospheres, for example, and the identification of potential threat materials in security applications. The high attenuation of the 60 GHz O2 allows high density unlicensed communication and covert systems.
Penetration of dust, smoke and fog can be achieved in the so-called ‘window’ frequencies where atmospheric attenuation is least and maximum range can be achieved. The window frequencies are best illustrated in Figure 3, which shows linear transmission at zenith with the contributions due to atmospheric gases and liquid water for a mid-latitude location. In the medical field terahertz (THz or sub-millimeter-wave) diagnostic tools use organo-metallic molecular signatures, which act as tracers for research in cancer bearing cell structures, and mm-wave ‘skin imaging’ passive scanners are under study for non-invasive, skin cancer diagnosis.

mm-wave Applications
Having outlined the background, consider the sectors in which millimeter-waves are being utilized. Of course, in a short article it is difficult to do justice to such a wide-ranging subject and apologies to the many researchers and manufacturers addressing topics that are too numerous to mention here.
An area to highlight is terrestrial and satellite communication systems and mm-wave ground-based communications. Cellular telephone and data link infrastructure is by far the largest commercial application of mm-wave systems. These use so-called ‘backhaul’ to provide wireless line of sight interconnections of the nodes in the system. In this way a radio network can be rolled out, to a large extent independent of existing infrastructure and is attractive for use in developing countries for that reason. A wide variety of manufacturers compete in the microwave/mm-wave radio market with the most common high capacity mm-wave radios using frequencies near 38 GHz.1
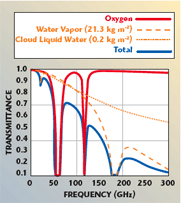
For even higher capacity still, frequencies in the 50 to 60 GHz band may be used at shorter ranges, for ‘hot spots’ and ‘last mile’ such as airports, railway stations and temporary set ups for broadcasting or special events. Companies such as Proxim Wireless2 offer high capacity point-to-point fiber-compatible radio systems for ranges of 0.25 to 1 km, operating in the unlicensed 57 to 64 GHz frequency band, as shown in Figure 4. Data rates depend on the modulation complexity and cover 125 Mbps to 1.25 Gbps. Standard interfaces such as OC3 (155 Mbps) and OC12 (622 Mbps) are used to interface point-to-point wireless networks.
Depending on the network, a mix of frequencies can be chosen in order to trade-off the required capacity, range and infrastructure cost. Although more sophisticated modulation formats have dramatically extended the capacity of a given link, the demand for further increases in bandwidth ensures a strong growth market for existing radios and also newly developing E-band radio-link products. E-band refers to the recent FCC licensed frequencies in the 71 to 86 GHz bands, which allow 1 to 10 Gbps wireless link operation over several miles. Applications include forming a bridge to fiber networks, and providing a backhaul channel for mobile and fixed wireless networks. Emerging E-band developments rely on the recent investments, improvements in performance and cost of suitable mm-wave integrated circuits.3

An additional factor in the emergence of commercially viable systems is the availability of suitable very high precision, low cost antennas. Higher gain is required for the greater ranges and is normally provided by precision parabolic reflectors. Companies such as Radiowaves Inc. have pioneered the manufacture and test of such higher frequency antenna solutions for digital radios. Precision antenna surface and feed position tolerances are required to generate tight patterns at mm-wavelengths, together with sophisticated antenna test ranges.4
Millimeter-wave Satellite Communications
To some extent, the deployment and development of mm-wave satellite communications systems mirrors those of ground-based systems. Highest volume applications involve the use of Ka-band (26.5 to 40 GHz) SATCOM terminals, which, in fact, use frequencies around 20 and 30 GHz for downlink and uplink, respectively. Conventionally the satellite transmits at the higher link frequency to take advantage of the easier deployment of the smaller sized antenna required for the same gain. The challenge for commercial ground terminal manufacturers is the integration of the MMIC device technologies into a high volume commercial application. This is largely a matter of quasi-automated production engineering, and design for production methodologies. An excellent reference paper describing the technology involved is that of R. Alm.5
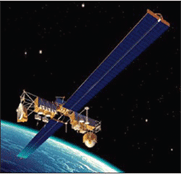
There is every reason to believe the use of higher frequencies will be commercially successful, given the service providers investment in Ka-band satellites, and the experience of current mass market product manufacturing, enabling successful direct broadcasting of TV in frequencies at Ku-band (10 to 14 GHz).
Additionally, given the importance of secure global communications for defence applications, higher data rate satellite systems have been deployed and are under development, some of which rely on frequency uplinks at around 44 GHz. An example is the US MILSTAR satellite network,6 which has been operational since 1994 and is being substantially upgraded (MILSTAR II, shown in Figure 5) to provide higher data rates (up to 1.5 Mbps for 192 channels), anti-jamming and inter-satellite cross links at 60 GHz. The most recent satellite in the constellation was launched in 2003.
Developments continue with MILSTAR ground terminals focussing on the use of high performance multiple frequency mm-wave converters and antenna systems including the manufacturing technologies required to guarantee a high level of reliability and lower cost using automated production methods (see, for example, a typical converter in Figure 6).
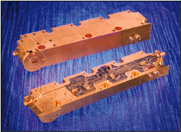
Scientific Applications of mm-waves
This subject is too vast to cover here, so just two examples are mentioned, where the contribution from mm-wave systems has been especially significant and developments rapidly evolving. First, millimeter-wave radio-astronomy has become a significant area of research over the last 30 years, since the first detection of molecular species with resonances in the mm-wave spectrum were discovered in extra-galactic molecular clouds. Today, ground-based millimeter and sub-millimeter instruments of increasing sophistication are being used as shared tools for the scientific community. Notable for its sheer scale is the Atacama Large Millimeter Array (ALMA), under construction in a high altitude desert site in Northern Chile.7
This millimeter and sub-millimeter radiotelescope is an interferometer consisting of 64 individual 12 m diameter dish antennas, each a wavelength coverage of 0.35 to 10 mm. Resolution will reach 10 milliarcseconds, an order of magnitude better than the Hubble Space Telescope, and it will be an imaging instrument. Scientific goals will be to investigate the cool universe that is radiation from materials at temperatures from 3 to 100 K, which give rise to spectral signatures in the sub-mm wavelength regions. This will allow the study of molecular gas and dust that forms building blocks for stars, planetary systems and galaxies.
Radio astronomy has historically driven technological developments in receivers, antennas and spectrometers at higher frequencies as the quest for more performance continues. Multi-channel imaging has become important in order to use the observation time effectively and this is driving the development of electronically scanned array sensors with expected spin-offs into other application areas.
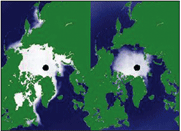
Also of note is millimeter-wave remote sensing, particularly satellite-borne remote sensing of the Earth’s atmosphere at millimeter wavelengths, which provides valuable information by global mapping from space. Atmospheric sounding is generally carried out by payloads covering frequencies of interest from around 10 to 183 GHz, designed to measure black body emission from the earth or planetary systems. Multi-spectral mm-wave radiometers have been carried on early satellites pioneered by NOAA-NASA in the 1980s. Such payloads normally are launched with and complement those used for operational meteorological instruments using multispectral infrared and optical imaging. Because land, water, air and ice have differing brightness temperatures, these features can be separated and in some cases uniquely identified.8 Figure 7 illustrates winter/summer ice pack coverage by microwave remote sensing.
Additionally, temperature sounding of the atmosphere can be provided by use of the properties of the 60 GHz oxygen resonances, and water vapour by means of measuring spectroscopic profiles. The spatial resolution tends to be low (commonly kilometers from spacecraft) and somewhat restricted by the available antenna aperture.
New developments aim at improving the scope in terms of the number of atmospheric constituents detected by increasing the range of frequencies detected, and by providing increased static coverage with geosynchronous satellites. One challenge in this regard with respect to geosynchronous spacecraft for earth imaging is the large antenna size (circa 2 to 3 m diameter) required for reasonable resolution, and the difficulty in scanning this large antenna to provide the required Earth ground coverage. Potentially, mechanical scanners of the type employed by mm-wave passive imagers (discussed later) may be a solution.
Millimeter-wave Radars
Largely developed from defence radar systems, commercial automotive radar systems using mm-wave sensors are now a reality. Initially based on Gunn diode technologies, it is becoming one of the main application areas for GaAs MMICs. Commercially available forward looking radar systems at 77 GHz allow detection at ranges up to about 200 m. These are marketed as autonomous cruise control (ACC) radars.9
To extend this functionality further requires significant improvements in performance (real time classification of targets with insignificant false alarm rates, for example). Despite this current limitation there is a strong market and competition among foundry providers to work with system designers and integrators.10 Most mm-wave foundries are working with the radar architects to fulfil the needs of the user, who ultimately is the automotive manufacturer. It is not usually cost effective to provide an ‘add-on’ package because of the high integration cost, so these radars need to be ‘designed in.’
GaAs technology promises a full solution to the mm-wave requirements but improvements are still needed (for example, in developing production level designs that do not rely on ‘post production’ tuning). At 77 GHz packaging is still challenging although MMIC manufacturers such as UMS have made considerable headway with multi-chip packaged approaches.
Another area that requires further development is the provision of suitable VCO solutions for FMCW radars. Initial 0.25 ?m PHEMT VCOs have been replaced with HBT designs that offer better low frequency phase noise performance and higher cut-off frequencies using a 0.15 ?m process; typically a creditable –85 dBc/Hz at 100 kHz offset can be achieved. As with most system related developments the challenge is to find the optimum combination of functionality and manufacturing cost that will support market growth.
Airport Radars

New developments aimed at improving safety following a number of high profile runway accidents (Concorde, Paris 2000 and Milan 2001) are making use of millimeter-wave radar technology. First, radar short-range sensors (~1 km) are radars placed on runway traffic aprons at strategic way points. This is a derivative of conventional approaches that illuminate the complete airport area with a single, high power downward looking radar.11 The advantages of this ‘cellular’ approach is that it can offer the possibility of automated collision warnings due to the reduced clutter and lower probability of false alarm. In addition, the lower power required for such sensors allows the possibility of significantly lower cost volume sensor production.12 Farran Technology manufactures a range of MMIC-based custom radar products, an example of which is shown in Figure 8.
Second, mm-wave foreign object detection radars for airport runway use have been successfully trialled13 and promise an increased level of safety by ensuring that operational runway debris is detected automatically using multiple millimeter-wave radar sensors. The radars can scan single or multiple runways and relay identified debris locations to a central user display. Automatic localised detection to within a few metres of a small object such as a metal bolt has been demonstrated under all weather conditions day or night. The radar developed by QinetiQ, UK, makes use of the high resolution available from a 1 m mm-wave antenna and the range provided by operating in a ‘window’ frequency of the mm-wave spectrum. Careful placement and calibration of the radars are required, however, in order to achieve full coverage and minimize local terrain effects.
Security Applications
The potential of mm-wave imaging systems to view concealed weapons or explosives has been the subject of intensive recent developments, responding to the security needs of airports and other establishments. Conceptually the mm-wave ‘camera’ is not new, having been prototyped by companies such as Millitech and others — notably TRW (now NGST10) — in the mid 1980s. However, market ‘pull,’ advances in mm-wave MMIC production technology, and new system architectures including mechanically scanned optics have spurred the latest developments.
Both passive and active technologies are in use, over a range of (loosely) mm-wave frequencies ranging from 24 to 140 GHz. At these wavelengths, fully populated or ‘staring’ arrays similar to current multi-pixel optical digital cameras are simply not feasible due to the high cost of individual receiver channels. Thus, the majority of systems use some form of mechanical scanning techniques to form an image from a reduced subset of receiver channels. The major cost element for such systems still tends to be the mm-wave front-end components or arrays. Lower frequency systems can be more cost effective due to the reduced device and packaging costs. However, a larger number of elements and active illumination is generally required to make up for the reduced resolution that is contingent upon the choice of frequency.

Advantages of higher operating frequencies include superior resolution for a given aperture size, enabling completely passive configurations with the requisite performance. Farran Technology has developed low cost advanced amplifier designs and arrays to support low noise imager systems. Advanced optical scanning techniques, such as those applied by the company’s patented designs, allow passive linear scans by a low cost multi-channel array to generate distortion-free mm-wave images at video frame rates (~10 Hz). This passive imaging camera (shown in Figure 9) is now manufactured by Smiths Detection,14 a division of Smiths Group plc. Given the sensitivity of the marketplace to the illumination of persons with millimeter-wave or any other radiation, there may be some advantages to this approach.
Enabling Technologies

It is probably already clear from the foregoing that key enabling technologies remain to further develop low cost high performance mm-wave MMIC devices, including device packaging techniques and the associated volume production methods. Of some interest in this trend towards lower cost is the potential impact of the reducing geometries and thus increased operating frequencies of CMOS circuits.
Although not designed specifically for analog mm-wave applications, there is potential for considerably lower cost CMOS finding its way into mm-wave applications.15 Also, considering the discrete device approaches of the late 1980s (see, for example, Figures 10 and 11) and today’s integrated circuit design equivalents, it is clear that technology has come a long way toward higher levels of integration, reduced size, weight and volume. Such developments allow for the use of increased complexity in demanding high reliability applications including commercial, space and defence markets, and ultimately lower costs for the application of mm-wave technologies in general.

Conclusion
The diversity of uses for the spectral region is what mainly characterises mm-wave applications. A few dominate where the commercial requirements have enabled significant investment in the technology area and there is no reason to suppose the future will be any different. The need for enhanced security or safety systems offers a significant and worthwhile challenge to the engineering of suitable passive or active mm-wave imaging systems either as standalone devices or in some situations as part of a suite of multiple detection strategies.
As demand for bandwidth increases the used radio communication spectrum looks certain to extend to upwards of 100 GHz, releasing products as spin-offs and reducing cost for new applications. Concomitant demand for power will drive increased developments in new semiconductor technologies areas such as gallium nitride, while demand for smaller devices and more efficient packaging will no doubt encourage the use of mm-wave MEMS. Emerging spectroscopic applications will require sensitive THz-based systems, and thus developments in new quantum sources and detectors will become important. As imaging sensors lower in cost, the deployment of electronically scanned systems in security, satellite systems and a host of civil applications will increase. Millimeter-wave applications have, by default, their particular niche areas that are propelled into the mainstream on commercial imperatives and it appears likely to remain so in the foreseeable future.
References
1. Alcatel, NERA, Nokia, Ericsson, etc.
2. Proxim Wireless, a division of Terabeam Inc.
3. Northrop Grumman Space Technologies, MMIC foundry (www.st.northropgrumman.com/velocium/products/e-band.html).
4. Radiowaves Inc.
5. R. Alm, Raytheon (www.csmantech.org).
6. NASA-JPL description of MILSTAR program (http://msl.jpl.nasa.gov).
7. Atacama Large Millimeter Array.
8. Satellite Remote Sensing.
9. United Monolithic Semiconductors.
10. UMS, Eudyna, NGST.
11. Raytheon ASMR radars.
12. Transtech-inc.com regarding airport intelligent radars.
13. QinetiQ UK, Tarsier FOD system.
14. Smithsdetection.com reference tadarvision web site.
15. IBM CMOS research (www-306.ibm.com CMOS.RF 130 nm process).
16. G.W. Petty, Atmospheric Transmission Chart: A First Course in Atmospheric Radiation, Sundog Publishing, Madison, WI.
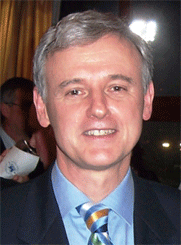
David R. Vizard
17. Applications developed by Farran Technology and now manufactured by Smiths Group.
David R. Vizard obtained his BSc degree in electronics from UMIST, UK, in 1968. He first worked for Marconi Communication Systems, UK, developing high linearity receiver front-ends. Later he joined the Radio Research Station, Ditton Park, where he initially researched mm-wave solar astronomy and low noise receiver technologies for astronomy and space borne applications. From 1978 he worked at the UK’s Science Research Council Rutherford Laboratories, Oxford, UK, in the role of group leader of the Millimeter-Wave Technology Division. In 1986, he took the role of technical manager at Farran Technology Ltd. Currently he holds a senior management position as director of technical sales, and over the years has published a wide range of papers related to developments in the millimeter-wave area. He currently directs Farran’s product development in numerous areas including passive imaging, space flight radiometers, millimeter-wave frequency extenders and EVS application radars using FMCW technologies among others.