Wireless local area networks (WLAN) are becoming increasingly popular in the corporate environment. They provide high speed connectivity and easy access to corporate networks without requiring wiring. Flexibility is key with WLAN capability.

In a corporate environment, individuals can be mobile and at the same time constantly be connected to their corporate network. This will allow a person to move to different parts of a building, download presentations from their corporate server and give presentations in different rooms. In a hotel setting, meetings can be held and groups can download presentations, surf the Web or easily download e-mail with WLANs. The ease of use, convenience of having no cords and affordable prices are making WLANs popular at home as well. The ease of use comes from the fact that anyone can deploy a WLAN network in a building. All that is required is an access point and a few WLAN cards and notebook PCs. One needs only to attach the access point to the server or PC, follow the installation instructions for loading the software on to the server and notebook with the WLAN card plugged into the notebook PC, and a WLAN is ready for use.
The main applications of WLANs are expected to be in the corporate environment, telematics (automotive), and hot spots such as hotels and airports. These public places experience a high concentration of users that demand mobile high data capability, and find difficulty in getting connected to wired locations. WLAN products offer better solutions and more flexibility to the end user than wireline or cellular modem. WLAN devices are relatively inexpensive and can offer better support for in-home distribution of DVD quality video, audio and other media applications.
This article discusses how RFICs with advanced architectures will play a key role in improving performance and lowering the cost of WLANs and other wireless systems.1 How the new standards will solve many of the current problems associated with wireless LANs, including speed, capacity, congestion, interference and security, is also considered. Novel multiband multimode WLAN products based on new IEEE 802.11g standards and innovative wireless ICs are discussed. Tradeoffs in designing multimode WLAN products, specifically devices operating under IEEE 802.11 a, b and g modes,2 are presented. This means that no matter where it operates, the WLAN card will identify the standard used at that location and will switch to the appropriate mode, IEEE 802.11a, b or g. This roaming methodology is similar to that of a cell phone, where the cell phone operates in multiple modes, such as analog or digital, and selects the appropriate mode for that location. This article focuses on some of the outstanding engineering challenges, and addresses issues regarding transceiver architecture and semiconductor process technologies.
WLAN Standards

WLAN standards developed by the IEEE 802.11 standards body have been evolving over the last few years. The Wireless Ethernet Compatibility Alliance (WECA) group is the organization that performs interoperability testing and certification. The benefits of using standards such as those published by the IEEE are countless. Compliance with the IEEE 802.11 standards makes it possible for interoperability between multiple-vendor appliances and the chosen wireless network type. This means that an 802.11-compliant access point can be purchased from one vendor and a PC card from another vendor while being assured that they will both interoperate within an equivalent 802.11 wireless network, assuming that the 802.11 configuration parameters are set correctly in both devices. Standards compliance also increases price competition and enables companies to develop wireless LAN components with lower research and development costs. Interoperability prevents dependence on a single vendor for appliances. Products having a WECA logo of wireless fidelity (Wi-Fi) identifies that the 801.11b WLAN cards from different vendors are interoperable. Similar WECA interoperability certifications are being developed for 802.11a and the upcoming 802.11g standard. Table 1 summarizes the various relevant wireless standards.
Most of the current WLAN products being deployed are based on the IEEE 802.11b standard operating at 2.4 GHz and are also certified by WECA as Wi-Fi-compliant products. The IEEE generated 802.11a standard is for WLAN devices in the 5 GHz band operating up to data rates of 54 Mbps. The IEEE 802.11a standard provides more bandwidth and channels than the 2.4 GHz band. In addition, the 5 GHz spectrum has less of the interference that plagues the 2.4 GHz band. The IEEE 802.11g standard is being developed to offer up to 54 Mbps data rates at 2.4 GHz using orthogonal frequency-division multiplex (OFDM) modulation. Development of the final IEEE 802.11g standard will take another three to nine months.
Overall, the use of OFDM at 2.4 GHz will increase the need for more linear PAs and high performance RFICs for that band. These events may slow down the 5 GHz demand a bit, but the 5 GHz band will continue to yield better data throughput because there are no legacy protocols to be accommodated, interference is minimal (no microwaves, portable phones or Bluetooth) and there are eight to 12 channels versus three for the 2.4 GHz band. The higher number of channels leads to an increased capacity for the 5 GHz band.
A chip set is being created that operates in multiple modes, that is IEEE 802.11 a, b and g, thus offering a migration path from the 11 Mbps 802.11b standard to the 54 Mbps 802.11a standard, allowing users to take advantage of the higher data rates and improved connectivity. This dual-band WLAN product will enable interoperability between high speed IEEE 802.11a-compliant networks operating in the 5 GHz band and current generation IEEE 802.11b-compliant networks operating in the 2.4 GHz band.
Why are Dual-band Multimode WLANs Important?
There are many benefits in using a multimode dual-band WLAN device. Since the 802.11b standard for wireless LANs was finalized in 1999, there has been explosive growth in the adoption of WLAN technology. Products compliant with 802.11b operate within the 2.4 GHz ISM band at data delivery rates of up to 11 Mbps, whereas 802.11a WLAN solutions operate in the unlicensed 5 GHz bands and can deliver higher data rates of 54 Mbps. This means that users and network managers are faced with a choice between 802.11a and b. Unfortunately, the choice is not always clear or easy and the result is often confusion.
Existing Investment and Migration
The rate of adoption and implementation of 802.11b networks is already considerable, taking advantage of reduced prices as the technology matures. The number of users and available access points is already significant, and maintaining backward compatibility will be important as users roam across a variety of networks.
Throughput
Networks compliant with 802.11b support a maximum throughput of 11 Mbps that is shared across all users. With a low number of simultaneous users, this may well be adequate for many of today’s applications. But with up to 54 Mbps offered by 802.11a, networks can also support streaming video or other such demanding applications. This makes it ideal not only for supporting future media networks, but also for supporting a larger number of simultaneous users. 802.11b cards are generally cheaper than 802.11a cards and are better suited for lower data rates and home applications.
Effective Range
There could be noticeable differences between the operating ranges of 802.11b networks at 2.4 GHz and 802.11a at 5 GHz. This derives from three aspects. First, radio propagation varies as a function of frequency. Second, since data rates are so much higher in 802.11a, the energy required to resolve the data is greater. Third, the physical properties of the network environment significantly affect the impact range. Taken together, the effective coverage area for an 802.11a network will likely be smaller than that for 802.11b. The range of 802.11g devices is expected to be higher than for 802.11a devices because of better propagation at 2.4 GHz, but 64 QAM OFDM modulation will reduce the range compared to the 802.11b devices due to higher vector error magnitude (EVM) and linearity requirements.
While 802.11b enables increased coverage from a single access point, its downside is that the 11 Mbps service is potentially shared by a greater number of users. Simply adding more access points is not necessarily a solution to deliver more bandwidth to the user because this causes potential interference problems as a result of overlapping coverage and the limited spectrum availability at 2.4 GHz. 802.11a has a smaller footprint, but can deliver a much higher bandwidth service to users in the coverage area. Adding additional access points does not create the interference issues experienced with 802.11b because the effective range is not as great and the number of available channels is greater in the 5 GHz band. The 802.11a standard will likely provide enhanced levels of service in areas of high user density, while 802.11b may still be an appropriate choice where lower user density and higher geographic coverage is required.
Benefits of Dual-mode
Just as cell phones operate seamlessly across various standards, it is believed that 5 and 2.4 GHz networks are complementary and will coexist for a long time. With the rapid adoption of Wi-Fi 802.11b networks, both users and operators require a migration path to 802.11a. Having to choose between 5 and 2.4 GHz is not appropriate since both bands have advantages and disadvantages. By delivering a cost-effective dual-mode solution, network managers can comfortably choose to deploy an appropriate mix of 5 or 2.4 GHz coverage throughout the area as the user profile dictates. The user will then be able to seamlessly roam throughout the coverage area, independent of the prevailing service, while accessing high data rate services where available.
Considering all the possible benefits of the multimode operation, a number of IC chipset providers are developing a set of WLAN ICs that will offer multiband and multimode operation just like the multimode cell did for the cellular market. Currently, the IEEE 802.11b standard at 2.4 GHz with 11 Mbps maximum speed is being deployed worldwide for WLAN application. However, as these WLAN networks become popular, the available 83 MHz bandwidth will not be enough to meet the needs of all the users. For higher data rates and higher capacity, IEEE 802.11g and IEEE 802.11a will offer good solutions. Many companies are already developing 802.11a products, which offer 54 Mbps maximum data rates and 200 MHz bandwidth in various 5 GHz bands. A LAN card using the IEEE 802.11a standard, however, does not work with an access point using the IEEE 802.11b standard. In fact, many large companies, like Microsoft, Compact and others, want these incompatibility problems solved and would prefer a multimode LAN card that operates in multiple bands. Such cards are not widely available at low cost today but the RFIC technology discussed here will make it a reality within the next few months.
WLAN System Design
Design considerations for a single-band 802.11a, b or g are somewhat simpler than for a multiband multimode WLAN since it is more difficult to meet all the specifications over multiple bands and still have low cost IC designs. Generally, the OFDM modulation used in 802.11a and g has tougher linearity and phase noise specifications. Also, the higher frequency “a” devices need higher frequency RFIC processes. The 802.11b specifications are the easiest from the radio perspective.
Dual-band Multimode WLAN System Architecture
A dual-band wireless LAN system architecture that allows operation in 802.11 a, b and g modes poses significant challenges. The problem becomes even more complex as one puts constraints that a tri-mode WLAN IC chipset must be only slightly higher cost than the existing 802.11a products.

The block diagram of such a multimode chipset is shown in Figure 1. These chips have state-of-the-art performance for 2.4, and 5 to 6 GHz band WLAN applications, addressing the IEEE 802.11b, 802.11a and g requirements in a single RF chipset. The chip yield and cost indicates that this dual-band multimode IC can be offered at only a slight premium over the single-band 802.11a IC solution. Higher levels of IC integration are planned for the near future to lower the costs even further.
In determining the appropriate transceiver architecture, cost, performance and form factor have emerged as the main selection criteria. Therefore, a thorough analysis of these criteria is the best method for qualifying the merit of the architecture for a dual-band WLAN transceiver. The dual-band WLAN system architectures can either use separate radios or share some components of the two-radio system to lower the cost. The proposed architecture shown here shares the IF chip, and the RF first down-conversion is done with separate chips.
There are cost, performance and risk tradeoffs between zero IF (ZIF) and dual-conversion architectures. ZIF architectures get rid of some of the filters that, if chosen carefully as in the proposed architecture, cost very little. These SAW filters are commonly used in cellular designs and are in volume production at low cost already. The other issue with the ZIF architecture is the risk and time-to-market. The traditional dual-conversion scheme has been chosen to minimize the risk and to get to the market quickly at low cost.
Some of the common problems inherent with the ZIF architecture are DC offset, flicker noise and LO pulling. DC offsets are mainly generated by the LO leakage which self-mixes, thereby creating a DC component in the signal chain that affects the receiver performance and can cause the baseband stages to saturate. Flicker noise, also known as 1/f noise, is a low frequency device noise that can corrupt signals in the receiver chain. Another concern with direct conversion is the pulling of the LO by the PA output mismatch variations, which affects the direct up-conversion process. This is because the high power output, which has a spectrum centered around the LO frequency, can disturb (pull) the transmitter VCO. Each of these issues can be addressed with careful design, and ZIF architectures have been successfully used in high volume cellular applications. However, the challenges are significant when you consider multiple bands and the extra performance required for the high data rate OFDM WLAN systems. This may require more IC design/fab iterations to resolve all these issues and meet all the WLAN system specs. Based on a cost, performance and risk analysis, it is believed that there will be a number of good ZIF and dual-conversion architectures deployed in the initial WLAN systems.

In today’s competitive environment, system design is not just a technical challenge but also a business strategy. In an environment where time-to-market, cost and performance are all critical to success, a thoughtful system design can save a great amount of development time and facilitate product evolution. Figure 2 shows a dual-band multimode WLAN chipset using the RF chips discussed here and a baseband Media Access Control/Physical Layer (MAC/ PHY) chip.
A combination of SiGe and GaAs technologies are used to achieve performance, cost and time-to-market benefits to address the WLAN RFIC market. These chips will provide a variety of complete chipset options when combined with a baseband MAC/PHY chip.
Integrated Cellular WLAN System
ICs that will allow easier integration of WLAN and cellular networks are also being developed. The new flexible transceiver architecture will enable wireless convergence of cellular (GSM/GPRS/WCDMA) and WLAN networks. This will give mobile users a single platform for traditional cellular voice communications, as well as truly high speed data communications using 802.11a, b and g standards.
The convergence of cell phone technology and WLAN technology will lead to an integrated mobile computing device (cell phone, PDA, notebook computer) that can communicate with both the existing cell phone infrastructure (for voice) and WLAN networks for high speed data applications.
At today’s throughput of 11 or 54 Mbps, WLAN technology will out-perform 2.5G or 3G in terms of deployment cost and data rates. However, the 3G networks will have wider coverage area compared to WLAN networks. A WLAN overlay, creating “hot spots” within the existing cellular infrastructure, is a quick and simple way for the carriers to begin offering competitive and truly high data rate services.
The IC architectures being developed for an integrated cellular/WLAN device will offer lower cost and higher performance than is achievable by having separate cell phone and WLAN cards. The performance improves in terms of device size, battery life and coverage area due to sharing of resources and use of advanced system architectures. There are significant design tradeoffs in designing RFIC for an integrated WLAN and cellular 2/3G system. Those will be discussed in a future article on that subject.
RFIC Design Tradeoffs
In this section, some of the RFIC’s design tradeoffs are highlighted, which designers have to consider when designing RFICs for wireless LAN applications. These are process technology tradeoffs, cost versus performance issues and single versus multiband architectures. Whether the dual-band WLAN products succeed will be determined predominantly by their cost compared to a single-band product. The dual-band WLANs may reach critical mass only if consumers are willing to pay the extra cost for them, relative to increasingly cheaper 802.11b systems.
RFIC Technology Choices
For wireless LAN application, the RFIC technologies must be chosen carefully to meet the challenging cost and performance objectives. In order to achieve the lowest cost solution, all of the RF circuitry must be integrated on as few high yield chips as possible. From the performance side, RFICs must be able to have high output power, high linearity and low current. Many IC technologies, including GaAs MESFET, HBT and PHEMT, as well as Si CMOS, SiGe BiCMOS and HBT technologies, are considered to obtain unique solutions to solve complex cost versus performance tradeoffs.
To perform an optimum WLAN chipset design, one needs expertise in a combination of skills, including RF system design, semiconductor process and IC design, packaging and antenna technologies. Traditional (silicon) semiconductor processes have difficulty supporting higher performance at higher frequencies and are useful only in very short-range data transmissions, such as the Bluetooth standard, popularized by silicon semiconductor companies.
Each of the SiGe, GaAs and InGaP3–5 processes have benefits for particular applications within the wireless markets. In determining the optimum process technology one needs to determine how to utilize the strengths of each process technology to meet the WLAN IC cost and performance objectives. One approach for a WLAN chipset is to utilize GaAs and SiGe technologies in a multichip module to offer high performance and a cost-effective, highly integrated, chipset solution. The time-to-market and risk are also important considerations in the decision process. Consequently, for a high performance front-end, a GaAs process is used for LNA, PA and switch components. For the up- and down-conversion either SiGe or GaAs processes can be useful. For a single-band WLAN solution, a CMOS process may be adequate for low performance levels, but for high performance multiband ICs, SiGe and GaAs may have an advantage over other technologies. This multichip module approach allows one to meet the performance, cost and time-to-market objectives with minimal risk.
The overall product cost is mostly attributed to the cost of semiconductor die, packaging and testing. For a complex IC such as a dual-band WLAN transceiver, where the die area is much larger than the single function ICs, die cost dominates these calculations and forces a move towards the low cost CMOS processes. On the other hand, the performance of the OFDM-based systems put a great demand on the performance (linearity, phase noise, etc.) of the transceiver, dictating a process equipped with high frequency bipolar capability. Therefore, the selection process should provide an appropriate balance between cost and performance if the development is expected to have a high probability of success. A combination GaAs/SiGe multichip module (MCM) solution with 90 percent of the functions in a low cost SiGe process offers a good compromise for many conflicting requirements.

Highly Integrated WLAN ICs
A complete chipset for a dual-band multimode WLAN card to meet IEEE 802.11a, b and g requirements is being developed. A combination of GaAs, MESFET/HBT, SiGe HBT and Si CMOS technologies are used to achieve high performance, low cost and quick time-to-market for the WLAN IC market. These ICs, coupled with baseband ICs, will form a complete chipset solution and a reference design for WLAN application. The HBT power amplifier technology offers higher power levels and linearity than is achievable with Si CMOS technology. Similarly, GaAs MESFET and PHEMT technologies offer high performance front-ends with low loss switches, PAs and LNAs with good noise figure. The competing Si CMOS technology cannot offer the performance these chips can offer. A photograph of a complete chipset assembled on an RF reference board is shown in Figure 3.
Power Amplifiers

In the power amplifier area, many unique and innovative solutions can be offered. For both WLAN and broadband wireless access (BWA) markets, as one goes to high data rates and higher modulation levels, the performance requirements for PAs increase in terms of linearity, efficiency and error vector magnitude (EVM). With the GaAs HBT and MESFET PA solutions, stringent efficiency and linearity requirements can be met to maximize throughput at high orders of digital modulation. With these solutions, the end user receives the maximum range, data rate and battery life simultaneously. Figure 4 shows examples of HBT power amplifiers. Figure 5 shows measured data demonstrating the performance of a 2.4 GHz HBT WLAN PA.

Multichip Module Technology
The MCM solution combines many IC process technologies in a single package to provide an optimized solution that lowers product cost and improves performance. The WLAN market asks for lower cost and better performance in the RF section. The best solution to meet these needs is to use compound semiconductor technologies such as GaAs for the higher performance RF section, and SiGe or CMOS for the lower performance RF or IF section. In order to meet the linearity and sensitivity requirements for this market, an LNA, front-end switch and PA may require a PHEMT process. An example of implementing this multichip module technology with a PA, LNA and switch is shown in Figure 6.

In order to combine multiple ICs in a MCM, various packages or substrate materials are used, such as PCB laminates, alumina, low temperature cofired ceramic (LTCC), glass and BT resin. Various passive components such as inductors, capacitors, resonators, filters, power combiners and splitters are developed using multiplayer ceramic substrates at very low cost. In this manner, 200 discrete passive components, such as inductors, capacitors, resistors, filters and antennas, can be replaced by a $0.20 LTCC substrate. Working with LTCC, however, requires significant experience in device modeling of 3D embedded passive components. In some products, the die cost is lowered by using semiconductor materials mainly to obtain the required active transistors. Most of the desired matching and filtering is done on a LTCC or soft board module, which has lower cost than the IC substrate material.
Figure 7 shows an example of MCM cost reduction, minimizing the use of expensive semiconductor materials, and using an LTCC substrate for passive components. Smaller size, lower cost and higher integration are maintained with this method.
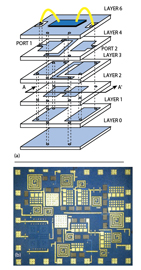
Future Technology Improvements
In the future, the trend for higher integration, coupled with clever architectures and proper mix of technologies, will lead to even lower cost and significant performance improvements in WLAN systems. As the WLAN costs continue to improve, these systems will work over a wider range using improved sectored antenna technology. Another interesting development to watch in the future is the integration of dual-band antennas, and dual-band front-end and transceiver modules. This further reduces cost and size, and improves overall system performance. Various wireless systems including WLAN, cellular, 3G and BWA systems will overlap over larger coverage areas. The user will be able to pick and choose which system he or she wants to use depending on their location. A number of promising technologies and the need for further integration levels will continue to push the need for RFIC designs and multichip module capability.
Conclusion
This article has shown that WLAN technology will have a key role in future wireless networks. Advances in IC technologies will lead to higher integration, lower cost and higher performance WLAN systems. This will enable low cost multimode, multiband wireless LAN cards, which will allow a WLAN card to operate in IEEE 802.11b, a or g modes. There are many design and process tradeoffs in developing these multimode WLAN cards. There is no single best approach, but there are a number of good architectures and approaches to designing these WLAN ICs with many tradeoffs. MCM technologies will allow integration of different ICs and passive components including inductors, capacitors, filters and antennas into a small package. These advanced IC and MCMs will also enable the convergence of cellular 2.5G and 3G networks with the WLAN network making PDAs and cell phones converge into a single device.
Acknowledgment
The authors would like to thank Pranav Patel, Vonda Thomas and Erica Travis for their help in preparing this manuscript.
References
1. S. Moghe, S. Han and C. Chun, “Semiconductor-based Transceivers for Broadband Wireless Customer Premises Equipment,” WCA Technical Symposium, January 2001.
2. Supplement to IEEE Standard for Information Technology, Part 11, “Wireless LAN Media Access Control (MAC) and the Physical Layer (PHY) Specifications, High Speed Physical Layer in the 5 GHz Band.”
3. B. Matinpour, C. Chun, S. Han, C.H. Lee and J. Laskar, “A Compact Monolithic
C-band Direct Conversion Receiver,” IEEE Microwave and Guided Wave Letters, Vol. 10, No. 2, February 2000,
pp. 67–69.
4. B. Matinpour, A. Sutono and J. Laskar, “A Low Power Direct Conversion Receiver Module for C-band Wireless Applications,” IEEE MTT Symposium Digest, May 2001, pp. 567–570.
5. C.H. Lee, S. Han, B. Matinpour and J. Laskar, “GaAs MESFET-based MMIC VCO with Low Phase Noise Performance,” IEEE GaAs IC Symposium, November 2000, pp. 95–98.
Sanjay Moghe received his MS degree in physics from IIT Bombay, India, in 1974, and his PhD degree in electrical engineering in 1980. Today he is president and chief technical officer of RF Solutions Inc. He has developed over 600 IC products and specializes in the design and development of very highly integrated, high performance RFIC systems. His previous work experience includes leadership roles at the broadband wireless engineering group of ADC Telecommunications and Pacific Monolithics. Dr. Moghe has published over 34 papers in the areas of wireless telecommunication systems, low noise and power amplifiers, microwave integrated circuit (MIC) and monolithic microwave integrated circuit (MMIC) design techniques.
Babak Matinpour is a staff engineer at RF Solutions Inc. where he leads a team of IC designers in the design and development of high performance SiGe RFICs for WLAN applications. Prior to joining RF Solutions, he participated in the design and development of SiGe RFICs with National Semiconductor, and K-band HEMT receiver ICs with Raytheon RF Components. Dr. Matinpour has published over 20 IEEE papers and has presented several conference presentations regarding compact and high performance GaAs and SiGe RFICs. He currently serves on the technical program committee for the MTT symposium.
Drayton Avera received his BS degree in electrical engineering from the Georgia Institute of Technology. Today he is the VP of business development for RF Solutions Inc. in Atlanta, GA. He has over 16 years of engineering experience developing RF and wireless products. Prior to joining RF Solutions, he held the position of director of engineering at LinkaNet Labs/DTS in Norcross, GA. Earlier in his career he held various engineering and management positions at both Motorola and NASA.