The Worldwide Interoperability for Microwave Access (WiMAX) system1 is becoming quite popular in allowing broadband wireless Internet access for private and business users. WiMAX is based on the IEEE 802.16 family of standards.2 Currently, the first system implementations, based on the 802.16-2004 specifications intended for fixed wireless access, are already being tested and even used.
The standard promises high transmission rates achievable by means of different modulation and coding schemes (up to 64-QAM-3/4) and using high gain antennas in a line-of-sight environment, if possible.

The first-phase deployment allows fixed access from home or office, with anticipated usage of indoor or outdoor subscriber antennas with quite high gain to attain the high signal levels necessary for high bit-rate transmission. The best choice should be subscriber antennas with a gain of approximately 20 dBi, mounted outside the building and oriented in the direction of the best signal reception. On the other hand, smaller antennas installed inside a building would be more suitable and sufficient enough in a higher cell-density environment.
Furthermore, proper reception direction could vary with time according to small-signal channel changes and thus require antenna orientation changes.

For these reasons, as a compromise to relatively high antenna gain, two different antenna prototypes meeting the small size requirements, indoor usage and direction adaptability were designed, built and tested for the two frequency bands planned for WiMAX use: 2.5 to 2.7 GHz and 3.3 to 3.6 GHz. These antennas have four beams covering four sectors in the horizontal plane. Each sector is covered by a vertical broadside array of two circular microstrip patches. The desired sector is selected by a microwave solid-state switch. This solution provides approximately 10 dBi of gain in an arbitrary direction in the horizontal plane and direction diversity at the same time. The vertical polarization of the antenna was selected at all times.
Antenna Design

To keep manufacturing costs down, it was decided to keep the antenna design as simple as possible. Microstrip patch antennas allow simple fabrication, impedance matching and polarization selection. A further reduction in manufacturing costs is obtained by punching the required microstrip structures out of a thin metal sheet. Using spacers and air as a dielectric, an improvement of the impedance bandwidth can be obtained at the same time.
The principles of operation of a circular microstrip patch antenna have been previously published.3 The basic radiation element consists of a circular metal disk, a so-called patch, spaced from the ground plane by a dielectric, as shown in Figure 1.
Such an antenna radiates from the side slot between the patch and ground plane, excited from the z-directed E-field distribution.
The feed point is offset from the patch center, determining the antenna impedance. The angular position of the feed point defines the orientation of the field distribution between the patch and ground plane and consequently the orientation of the achieved linear polarization.
The antenna resonant frequency depends upon the patch dimensions.
For a required resonant frequency fr of the dominant mode TM11, the patch radius can be approximately expressed as3

where ae is the effective radius, which is slightly larger than the physical radius, a, due to fringing-field effects at the patch edge. The physical radius, a, can be obtained, using iterations from the relation

Patch antennas are often made, using a microstrip technique, on usually quite thin dielectric substrates of various dielectric constants (εr > 2). Higher substrate permittivity results in a narrower impedance bandwidth and lower radiation efficiency.3 Consequently, the decision was made to design an antenna on an air substrate (εr∼1) with the patch and ground plane made from a thin brass sheet and separated by a spacer of height h. Standard metal M3 screws were used as spacers in the patch center where the electric field is close to zero.
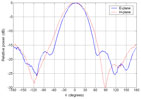
Due to the low dielectric constant of the air, a better radiation efficiency and a wider impedance bandwidth were achieved.
The basic design of a single linearly-polarized circular patch on a square ground plane results in quite similar E- and H-plane radiation patterns, as shown in Figure 2, and has a gain of typically 8.5 dBi, which is less than the desired 10 dBi. To achieve a higher gain, the beamwidth was narrowed in the vertical plane (E-plane in the case of vertical polarization).
A narrow vertical beamwidth is not critical for the proposed WiMAX network. On the other hand, the horizontal beamwidth should remain the same to cover the desired sector.
There are various possibilities to narrow the radiation pattern in the vertical plane.
Three such possibilities are shown in Figure 3: (a) to enlarge the ground plane surface in the vertical direction; (b) to introduce λ/4 flaps on the top and bottom edges of the ground plane; (c) and/or to stack two patches vertically on the same ground plane.

The first two solutions did not provide enough gain improvement in the available space. On the other hand, the last solution provides enough gain using a simple series-feed network, connecting the two patches with a λ/2 line.
The characteristic impedance of the connecting line is irrelevant due to the λ/2 line-length and does not affect the antenna operation.4 In the solution adopted, the patches were stacked at a distance of 0.8λ between the patch centers.
The vertical-plane radiation pattern of such an array results in a higher antenna gain, up to 12 dBi, as shown in Figure 4.
Such an antenna design is convenient for the desired purpose: it has a sufficiently wide radiation pattern in the horizontal plane to cover a wide horizontal range and a moderately high gain on account of the narrower radiation pattern in the vertical plane.
The described two-patch array covers one 90° horizontal sector. Four such arrays are used to cover the whole 360° range.
The desired sector is selected by a solid-state RF switch. The complete antenna design is shown in Figure 5.

Its dimensions were obtained using theoretical calculations first and then final experimental optimization.
Switching Circuit
The described four-sector antenna should allow signal reception and transmission in the most suitable direction without physically turning the antenna. A combining network, including adjustable phase shifters and variable-ratio power splitters, was found to be too complex. A simple and efficient solution is a SP4T switch selecting one of the four two-patch arrays.

The same switch also provides direction diversity in the case of a dynamically changing propagation channel. An integrated circuit HMC241QS16 (manufactured by Hittite) was used as a solid-state SP4T switch.
The packaged device was installed on a small microstrip board etched on a 19 mil-thick Rogers R4003 laminate. A cheaper version, on FR4 laminate, gave a 0.5 dB higher insertion loss. The microstrip board also carries the DC decoupling capacitors and the logic-level converters for the two control inputs A and B of the solid-state switch.
The electrical circuit diagram is shown in Figure 6. The whole board operates from a single supply voltage ranging between +5 and +6 V. The microstrip board fits in the space in the center of the four two-patch arrays so that no additional RF connecting cables are required, as shown in Figure 7.
The lengths of the 50 Ω microstrip lines connecting the HMC241QS16 IC to the four two-patch arrays were carefully selected to compensate for both antenna and switch impedance imperfections. Besides the 50 Ω coaxial cable, there are four wires connected to the antenna: the two switch control signals A and B, the switch supply voltage, and ground.
The sector-selection logic is not integrated in the antenna; the two control signals A and B are provided by an external circuit. Photographs of the final antenna prototype are shown in Figure 8. As a final product, the antenna is integrated in a plastic housing with an input/output RF connector, a DC connector for the power supply and control voltages and four LEDs, indicating the active antenna sector. The effects of the plastic radome, made from 4 mm thick dielectric with a relative permittivity in the range between 2 and 3, were taken into account in the antenna design.

Antenna Characteristics
The described antenna was fabricated in two different versions, for two different frequency ranges centered at 2.60 and 3.45 GHz. The impedance matching of both fabricated prototypes provided a return loss (S11) better than –12 dB over the entire operating frequency ranges of 2.5 to 2.7 GHz and 3.3 to 3.6 GHz, as shown in Figure 9.
The radiation patterns for the 2.6 GHz prototype antenna have been measured. The radiation patterns for all four antenna sectors in the horizontal plane (H-plane) and for a single sector in vertical plane (E-plane), measured at the center frequency, are shown in Figure 10.
The 3 dB beamwidth is approximately 30° in the (vertical) E-plane and 65° in the (horizontal) H-plane.
At the ±45° sector overlap, the horizontal radiation patterns decrease by approximately 5 dB. The 3.45 GHz prototype patterns are similar and are omitted for brevity.
As shown, all two-patch arrays in the group exhibit almost equal radiation patterns, with slight differences due to the manufacturing tolerances.
The radiation patterns remain similar over the whole specified frequency range of 2.5 to 2.7 GHz or 3.3 to 3.6 GHz. The frequency dependence of the radiation patterns is shown in Figure 11.

The final antenna gains, including radome and switch losses, are 11.5 dBi for the 2.6 GHz version and 9.5 dBi for the 3.45 GHz version, in the direction of maximum radiation.
The gain difference, of approximately 2 dB between both versions, can be explained by the higher losses in both the solid-state switch and the microstrip interconnection circuit at the higher frequency.
Conclusion
The presented antennas were designed and built as part of the WiMAX subscriber station deployment within the framework of the WiMAX system implementation by the company Telsima.
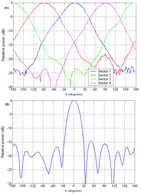
All of the prototypes built met the system requirements: good impedance matching and moderate gain in the 10 dBi range, within the specified dimensions of the radome.
The prototypes are also quite inexpensive to fabricate in production volume. Although the design was tested at two frequencies, the antenna can easily be redesigned for other frequency ranges as well.
References
- WiMAX Forum (www.wimaxforum.org/home).
- IEEE 802.16 LAN/MAN Broadband Wireless LANS (standards.ieee.org/getieee802
802.16.html). - R. Garg, P. Bhartia, I. Bahl and A. Ittipiboon, Microstrip Antenna Design Handbook, Artech House Inc., Norwood, MA, 2001.
- T.A. Milligan, Modern Antenna Design, Second Edition, John Wiley & Sons Inc., Hoboken, NJ, 2005, p. 329.

Tomaz Korosec received his BSEE degree from the University of Ljubljana, Slovenia, in 2005. Since October 2005, he has been working as a junior researcher in the radiation and optics laboratory, faculty of electrical engineering, University of Ljubljana, where he is doing his PhD thesis. His research interests include RF and optical communications and phenomena.
Patrik Ritosa received his BSc degree in electrical engineering from the University of Ljubljana, Slovenia, in 2004. He is currently a junior researcher in the radiation and optics laboratory, faculty of electrical engineering, University of Ljubljana, where he is working on his PhD degree. His research interests include fiber optics communications and adaptive antennas.
Bostjan Batagelj received his PhD degree from the University of Ljubljana, Slovenia, in 2003. He is currently a senior lecturer on the faculty of electrical engineering. His research interests include RF and optical transport systems.
Matjaz Vidmar received his BSEE, MSEE and PhD degrees from the University of Ljubljana, Slovenia, in 1980, 1983 and 1992, respectively. He is currently teaching undergraduate and postgraduate courses in electrical engineering at the University of Ljubljana. His current research interests include microwave and high speed electronics, ranging from avionics to optical fiber communications.