With the growing prevalence of low-power sensor networks, simultaneously powering many devices is an increasingly important topic. The conventional way of powering devices (e.g., with cables or batteries) is becoming impractical with the increasing device density in smart buildings. Cables limit mobility, and batteries require additional maintenance because they must be periodically exchanged. Wireless power transfer (WPT) at a distance and energy harvesting (EH) enable low-power devices to operate indefinitely without requiring maintenance and allowing for greater mobility range.
While the physical concepts underlying WPT and EH have already been thoroughly studied, the use of RF waves to transmit energy has not been widely adopted due to challenges such as low end-to-end efficiency and a lack of coordinated effort to ensure interoperability. This article addresses these challenges by investigating the impact of the waveform on RF-to-DC conversion efficiency, confirming that high peak-to-average power ratio (PAPR) signals can increase efficiency in rectifier circuits. It also provides an overview of current standardization efforts and demonstrates how a prototype test setup from Rohde & Schwarz is contributing to the development of WPT technology. Furthermore, it discusses the potential of shifting to higher operating frequencies to enable smaller, more efficient devices and unlock the mass market potential of WPT. The reported findings offer valuable insights for researchers and industry stakeholders working to overcome the technical and practical hurdles in WPT and EH.
HISTORY OF WPT AND CURRENT CHALLENGES
The fundamental concept of WPT dates back to the 19th century. In 1888, Heinrich Hertz succeeded in experimentally verifying Maxwell’s prediction of electromagnetic waves, confirming the possibility of transmitting power wirelessly from one point to another. In 1899, Nikola Tesla envisioned transferring considerable amounts of electric energy globally. To this end, he built the Wardenclyffe Tower, which had the goal of transatlantic wireless communications and power transfer.
No further progress was made in far-field WPT for several decades until advancements in microwave technology in the 1960s. William C. Brown pioneered the modern era of far-field WPT. He demonstrated the wireless powering of light bulbs and electric fans with a 5.5 m distant power transmitter (Tx). He also demonstrated the first microwave-powered helicopter. Another driving application for RF WPT was the solar-powered satellite. In this system, solar energy from the sun is captured by a geostationary satellite. The energy is converted in space into microwave power, which is beamed to Earth and converted to DC power. These experiments on far-field WPT focused on high-power RF transmissions over long distances using large antennas.
The recent proliferation and ubiquity of low-power communication devices, such as wireless sensors, IoT devices and wearable electronics, has drawn interest to using WPT for low-power delivery (e.g., microwatt to milliwatt) over short to moderate distances of a few meters. Despite the existing research on WPT, significant efforts are still required to model and optimize the receiving circuits to achieve the highest conversion efficiency possible. Another issue is coordinating development efforts and products to ensure coexistence and interoperability between WPT devices.
RF-to-DC Conversion Efficiency
At its core, the RF-to-DC conversion process is relatively simple; only two components are involved, namely the antenna and the rectifier, commonly grouped under the name “rectenna.” The antenna receives the RF signal, and the rectifier converts it to DC. The rectifier typically contains diodes followed by a capacitor-based lowpass filter (LPF), making it a nonlinear circuit element.
A high RF-to-DC conversion efficiency has clear advantages. First, an efficient WPT means that less energy is wasted as heat. This improves the overall system performance, including the power transfer range and device charging times. Second, high efficiency allows for higher received power, expanding the possible areas where WPT can be deployed.
Theoretical Considerations
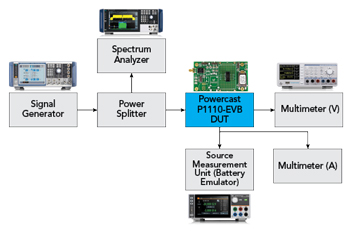
Figure 1 Setup for waveform efficiency comparison.
The rectifier component of an RF-to-DC converter is a nonlinear circuit element due to the relationship between the circuit’s RF input power and DC output power. The waveform has an impact on the conversion efficiency.1 Signals with high PAPR result in higher harvested DC power. This is because the peaks of a high PAPR signal are more likely to exceed the turn-on voltage of the diode compared to a constant envelope signal with the same average power but lower peak power. In addition, the peaks of a high PAPR signal can charge the capacitors to a higher voltage level. For an output LPF with a long time constant, the capacitor can maintain the charged voltage level until the next signal peak.
Waveform Efficiency Comparison
To compare the effect of high PAPR signals on the RF-to-DC conversion efficiency of a prototype board, a signal generator is connected to a power splitter, which equally divides the incoming power between the spectrum analyzer and the RF-to-DC receiver (Rx) board from Powercast. A battery emulator is connected to the DC output of the Rx. This eliminates the need for a physical battery by emulating the behavior of various battery types, including Li-Ion and NiCd. The states of charge, current and voltage at the battery terminals are also displayed. Precise measurements of both voltage and current are conducted using high precision multimeters. The setup is controlled remotely such that tests and other evaluations run automatically. Figure 1 shows the setup diagram with the devices listed in Table 1. The measurement parameters and configuration can be taken from Table 2.
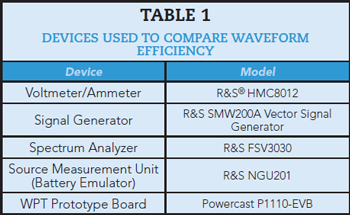
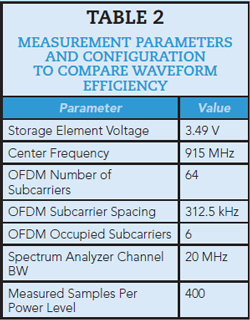
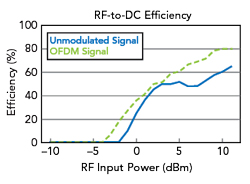
Figure 2 RF-to-DC conversion efficiency of a prototype board.
Figure 2 shows the RF-to-DC conversion efficiency for different RF power inputs to the Rx circuit. The OFDM signal generally outperforms the sinusoidal signal throughout the input power range. This confirms the hypothesis that high PAPR signals lead to better rectifier efficiency because of their nonlinear nature. Another interesting observation is that the higher efficiency of the high PAPR signals decreases the minimum input power required by the rectifier by about 2 to 3 dB. In other words, the RF-to-DC converter sensitivity improves with high PAPR signals. This translates to greater operational ranges, higher output power levels and a better user experience.
3GPP and Ambient IoT
Interoperability is a crucial factor in future WPT systems. It enables wireless device charging without compatibility issues. This leads to a more diverse ecosystem that promotes market growth and innovation, eventually making WPT technology more affordable.
The 3GPP, a global standards organization for telecommunications, has traditionally focused on communication standards for 3G to 5G networks. However, their existing standards did not previously address the specific needs of low-power, low-complexity devices that may operate without batteries and consume less than 10 mW of power. Recognizing this gap, 3GPP has introduced the concept of “Ambient IoT” in their recent studies, outlining requirements and air interface specifications in TR22.840 and TR38.848. The low-power nature of Ambient IoT devices makes them ideal candidates for RF-powered operation. In Release 19, the 3GPP Ambient IoT initiative tackles key aspects such as use cases, connectivity architectures, spectrum allocation, EH and coverage, paving the way for innovative IoT applications.
AirFuel Standardization Efforts and Testing
For a technology to be available for commercial use, it is essential to have a standard that can be applied to devices from different manufacturers. Moreover, it is necessary to define compliance testing requirements that must be met before a device can be deployed in the market. This is important for ensuring that a new technology like WPT has no negative impact on existing technologies. To this end, AirFuel Alliance (AFA) has defined a standard for RF WPT.
AFA is a global coalition of companies developing standards for wireless charging. AFA defined the AirFuel Resonant standard that uses magnetic resonance at 6.78 MHz in the industrial, scientific and medical (ISM) band to charge devices with powers up to 50 W at distances up to several centimeters. AFA also defined the AirFuel RF standard for using RF signals in the 900 MHz ISM band (865 to 868 MHz and 902 to 927 MHz, depending on the region) to deliver up to 1 W of wireless power over distances of several meters. This provides the Rx with motion freedom while it is being powered. AirFuel RF uses Bluetooth® Low Energy for the control channel signaling between the Tx and Rx. Receiving devices can establish a full Bluetooth connection to the Tx or exclusively broadcast Bluetooth advertisements to request that any Tx in range send them power.
The AirFuel RF standard Baseline System Specification (BSS) was released for AFA members in January 2023. The Interoperability (IOP) and Conformance Test Specification (CTS) were released for AFA members in April 2024. These standardization efforts aim to make RF signals for wireless charging as ubiquitous as Wi-Fi for communications.
As a member of the AFA, Rohde & Schwarz contributes to developing WPT. The Rohde & Schwarz WPT Project targets the emerging WPT market by leveraging the capabilities of its existing test and measurement equipment to provide a comprehensive test solution for wireless power Tx and Rx. The involvement of Rohde & Schwarz is key to refining the testing procedures of AirFuel RF.
The Rohde & Schwarz WPT Project created a test setup based on Figure 1 to deliver precise and actionable feedback during the AirFuel RF standard development process. A software framework complements the hardware, allowing precise control of individual devices. It also allows tests to be orchestrated through an API, command line interface and user-friendly web interface. Apart from testing the general capabilities of the device under test (DUT), the setup implements the AirFuel CTS for the Rx.
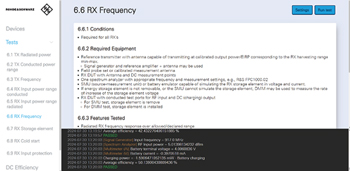
Figure 3 Test setup web-UI including AirFuel Conformance Test Specification.
Figure 3 is a screenshot of the web interface showing one of the AirFuel RF CTS tests, namely the “Rx Frequency” test. This test case evaluates the DUT frequency range under various power levels. As shown in the black window in Figure 3, the signal generator feeds the input frequencies to the DUT at different power levels. It is verified whether the device charges for each frequency and power level combination. A test is successful if the device charges under the specified conditions; otherwise, the test is considered failed. The user can configure the test parameters, launch the test and monitor the progress within the interface.
TECHNOLOGY OUTLOOK
Several technological developments are expected to shape the future of WPT and EH. One key trend is the shift toward higher frequencies, which promises advantages such as smaller form factors and higher power transfer rates. However, this development raises concerns about safety, cost and system complexity.
Higher Frequencies
Despite the associated higher path loss, there is an ongoing transition to higher operating frequencies.2 Currently, some WPT efforts are focused on GSM bands and sub-GHz frequency ranges. The literature and latest market trends show a clear preference toward the ISM bands, especially in the 2.4 and 5 GHz ranges, but also ranging up to 60 GHz. To understand this interest, it is necessary to consider the numerous advantages that higher frequencies provide.
Smaller Antennas and Devices
It is well known that the size of an antenna is intrinsically related to the wavelength of the radio waves it transmits or receives, with λ/2 being the optimal antenna size for resonance and maximum efficiency. By increasing the frequency and, therefore, reducing the wavelength, antennas can be made smaller and more practical for mobile IoT devices. Early attempts at WPT required very large antennas because it was extremely difficult to generate higher frequencies then.3 For example, utilizing DTV frequencies (470 to 770 MHz) for EH is difficult due to the impractical antenna size.4
Antenna components in the 2.4 GHz range, for example, are in the centimeter range. These can be developed based on well-known designs. For transmission at longer distances, this frequency range exhibits an excellent compromise between propagation attenuation at higher frequencies and the larger dimensions necessary for transmission at lower frequencies.3
Improved Beam Shaping Capabilities
Apart from their size, smaller antennas also offer beam shaping benefits. Precise beam shaping on higher frequencies while maintaining moderate antenna sizes is key to the widespread use of WPT.3 A sharper beam is highly advantageous because it leads to higher antenna gain, which increases the power delivered to the Rx. This effectively increases the range and counteracts the higher path loss at higher frequencies. Extending the transmission distance proportionally is possible by utilizing transmit antenna arrays with more elements or operating at higher carrier frequencies. For example, transitioning from 2.4 to 60 GHz could theoretically increase the range by a factor of 25.5 However, possible tradeoffs must be considered. Most importantly, the conversion efficiency decreases with increasing operating frequencies6 because of some circuit topologies’ lowpass characteristics. Addressing these challenges is crucial for the future of WPT, as large arrays of steerable antennas, capable of delivering narrow beams with high gain, have the potential to enable widespread adoption of WPT over long distances.7
Radiation Safety Concerns
Although traditional communication systems do not pose any radiation risk to the users, the higher power density of WPT systems must be closely studied. The narrower the transmission beam, the higher the power density, which can quickly surpass the radiation limits for humans, as stated by organizations such as the FCC. This is especially true in the case of very narrow beams that are achievable at high frequencies and are necessary to achieve high antenna gain. This work5 highlights the importance of considering the beamwidth to determine the unsafe beam interception distance. In addition, these calculations depend on local regulations applicable to the frequency ranges, exposure times and duty cycles of the signal.8
Increasing the operating frequencies of WPT devices makes more precise beamforming possible at the expense of higher radiation risks for humans and animals. This can be circumvented by implementing systems that steer the beam away from living organisms. However, such a system is a significant technical challenge. Another approach proposed by Ossia9 is the use of retrodirectivity to identify all safe paths between Tx and Rx. This would be done using beacon signals before power transmission. For now, multiple open questions remain about the safety mechanisms for radiative WPT.
CONCLUSION
WPT and EH are not new concepts. However, there are significant challenges when it comes to implementing this technology. Key challenges are low end-to-end efficiency and industry coordination to ensure interoperability of these wireless systems. For the technology to advance, it is crucial to understand how to improve the RF-to-DC conversion efficiency by transmitting high PAPR signals. Ongoing standardization efforts, which Rohde & Schwarz actively supports with its prototype test setup, are paving the way for commercialization. A shift toward higher operating frequencies would enable narrower transmission beams and allow for smaller devices. This may play a decisive role in WPT technology entering the mass market. However, significant optimization is still required to overcome the decreasing efficiency of most rectifier circuits at higher frequency ranges.
References
- A. Boaventura, A. Collado, N. B. Carvalho and A. Georgiadis, “Optimum Behavior: Wireless Power Transmission System Design Through Behavioral Models and Efficient Synthesis Techniques,” IEEE Microwave Magazine, Vol. 14, No. 2, 2013, pp. 26–35.
- M. U. Hoque, D. Kumar, Y. Audet and Y. Savaria, “Design and Analysis of a 35 GHz Rectenna System for Wireless Power Transfer to an Unmanned Air Vehicle,” Energies, Vol. 15, No. 1, 2022.
- W. C. Brown, “The History of Wireless Power Transmission,” IEEE Transactions on Microwave Theory and Techniques, Vol. MTT-32, No. 9, 1984, pp. 1230–1242.
- C. Mikeka and H. Arai, “Design Issues in Radio Frequency Energy Harvesting System,” 2011.
- K. Huang and X. Zhou, “Cutting the Last Wires for Mobile Communications by Microwave Power Transfer,” IEEE Communications Magazine, Vol. 53, No. 6, 2015, pp. 86–93.
- C. R. Valenta and G. D. Durgin, “Harvesting Wireless Power: Survey of Energy-Harvester Conversion Efficiency in Far-Field, Wireless Power Transfer Systems,” IEEE Microwave Magazine, Vol. 15, No. 4, 2014, pp. 108–120.
- N. A. Muhammad, N. Seman, N. I. A. Apandi and Y. Li, “Energy Harvesting in Sub-6 GHz and Millimeter Wave Hybrid Networks,” IEEE Transactions on Vehicular Technology, Vol. 70, 2021, pp. 4471–4484.
- I. Prlic, J. Sisko, V. M. Varnai, L. Pavelic and J. Macan, “Wi-Fi Technology and Human Health Impact: A Brief Review of Current Knowledge,” Archives of Industrial Hygiene and Toxicology, Vol. 73, 2022, pp. 94–106.
- “Unwired: The Blog,” Ossia, Web: www.ossia.com/blog.
Further Reading
K. Detka and K. Górecki, “Wireless Power Transfer - A Review,” Energies, Vol. 15, 2022, p. 7236.
D. Pavone, A. Buonanno, M. D’Urso and F. Della Corte, “Design Considerations for Radio Frequency Energy Harvesting Devices,” Progress In Electromagnetics Research B, Vol. 45, 2012, pp. 19–35.
S. Ladan, A. B. Guntupalli and K. Wu, “A High-Efficiency 24 GHz Rectenna Development Towards Millimeter-Wave Energy Harvesting and Wireless Power Transmission,” IEEE Transactions on Circuits and Systems I: Regular Papers, Vol. 61, No. 12, 2014, pp. 3358–3366.
N. A. Muhammad, N. Seman, N. I. A. Apandi and Y. Li, “Energy Harvesting in Sub-6 GHz and Millimeter Wave Hybrid Networks,” IEEE Transactions on Vehicular Technology, Vol. 70, No. 5, 2021, pp. 4471–4484.