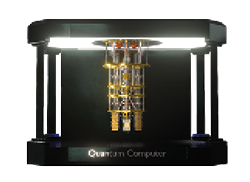
Figure 1 A superconducting quantum computer.
The expansive realm of quantum information science and technology (QIST) encompasses quantum computing, communication, sensing and simulation. These groundbreaking technologies are poised to rapidly reshape our world. Aspects like secure quantum communication, quantum internet and advanced quantum sensors are on the horizon, set to become integral parts of our daily lives. Quantum computing, however, poses significantly greater challenges and requires more time to realize its anticipated potential. Figure 1 illustrates a superconducting quantum computer.
The QIST field is still emerging. As the discipline continues to develop, it will present numerous technical challenges alongside the demand for a highly skilled workforce. A significant part of this challenge lies in cultivating a multidisciplinary workforce that combines excellent analytical skills with specialized expertise in engineering and science.

Figure 2 (a) Photon emission as an electron relaxes to a lower-energy orbit. (b) Hydrogen atom Balmer series transitions. (c) Quantum harmonic oscillator with equidistant energy levels. (d) Anharmonic quantum oscillator.
Hughes et al. highlight the quantum industry’s urgent demand for skilled professionals in Assessing the Needs of the Quantum Industry.1 Conventional quantum engineering training tends to be a prolonged process, posing a challenge. However, by prioritizing essential real-world technical skills crucial for quantum research and development, the training process can be accelerated. Furthermore, there is a significant opportunity to upskill current professionals, including hardware and software engineers, to meet the increasing demands of the QIST field. To expedite this transition, having resources that facilitate rapid training is crucial. Recognizing this need, companies like Quaxys are specializing in practice-oriented courses for specialized microwave and quantum hardware training. These efforts have also spawned books, such as Microwave Techniques in Superconducting Quantum Computers2, that aim to impart essential skills for quantum hardware engineers involved in the development of semiconductor quantum platforms, covering topics like superconducting, spin and topological qubits.
Quantum computing platforms fall into two categories: natural systems like atom and ion qubits and artificial systems like superconducting, spin and topological qubits. Each category demands specific skills, some unique to the platform and others shared. The following sections investigate the four primary skill sets: microwave engineering, cryogenic engineering, nanofabrication and data acquisition and measurement that are crucial for quantum hardware engineers and companies working on semiconductor qubits to enable success in this field.
MICROWAVE ENGINEERING
The realm of quantum technologies, particularly semiconductor qubits, has opened numerous possibilities for hardware engineers specializing in microwave and embedded hardware engineering. As a starting point, it is instructive to explore some of the fundamentals. These include the definition of a qubit and the rationale behind utilizing microwave frequencies for superconducting qubits.
A qubit is a fundamental unit of information in quantum computing, representing a two-level quantum system. The encoding of information occurs through the ground state, represented as “0” and the excited state, represented as “1.”
In the following, we conceptually describe how a superconducting qubit can be implemented as a nonlinear LC circuit to mimic the behavior of an atom. Figure 2a illustrates an atom relaxing from a higher-energy level to a lower one. The characteristic of this transition is the emission of a photon with a certain frequency corresponding to the difference in energy levels, as shown in the diagram. In an atom with more than two levels, such as the hydrogen atom, a series of spectral emission lines result from an electron transitioning from a higher-energy orbit to a lower-energy orbit. These are called Balmer series transitions of a hydrogen atom and are shown in Figure 2b. The n values are integers corresponding to the principal quantum numbers involved in the transition. Each transition has a unique frequency, as shown. It’s possible to mimic this atomic behavior using a superconducting circuit.
A superconducting LC circuit can be shown to be a quantum harmonic oscillator with equidistant energy levels, as illustrated in Figure 2c. Consequently, there is no unique transition frequency between energy levels, making it impossible to isolate and address only two energy levels to build a qubit.
By adding non-linearity to the LC circuit using a Josephson junction, we can build an anharmonic quantum oscillator with non-equidistant energy levels, as shown in Figure 2d. This results in unique transition energies between energy levels, similar to a real atom. This type of nonlinear superconducting circuit provides a two-level system with a unique transition frequency, enabling the construction of a qubit.
Consider the scenario where the energy difference between the ground and excited states is low, allowing thermal energy in the qubit’s environment to cause the qubit to transition from the ground state to the first excited state. This uncontrolled thermal transition is undesirable since we seek precise external control over the qubit’s state.
Semiconductor qubits are housed within a dilution refrigerator for various reasons. Foremost among these reasons is the need to mitigate uncontrolled excitation caused by thermal energy. The thermal energy can be calculated in Equation 1:
Where:
kB = Boltzmann’s constant
T = temperature
A rough calculation provides insight into the possible effects of unwanted thermal energy. The thermal energy calculated in Equation 1 will have an associated frequency. The Planck-Einstein relationship of E = hf can be used to determine the frequency (fth) associated with thermal energy in a dilution refrigerator. This is shown in Equation 2:
Where:
h = Planck’s constant
A typical dilution refrigerator can achieve a temperature of 20 mK, corresponding to a frequency of fth = 0.4 GHz. To minimize the occurrence of thermal excitations from the ground to the excited state of the qubit, it is essential for the qubit’s transition energy (E01) to be much higher than the thermal energy, i.e., E01 >> Eth. The term “much higher” implies at least 10x greater, which leads to a transition frequency f01 = 10fth = 4 GHz.
Most superconducting charge qubits, such as the transmon, operate in the microwave frequency range of 2 to 10 GHz. Microwave frequencies are particularly appealing since they are sufficiently high to leverage standard cryogenic and well-established microwave techniques and components commonly used in the telecommunications industry. While higher frequencies may offer certain advantages, they pose challenges regarding component cost, design complexity and fabrication capabilities. Therefore, to effectively design and operate superconducting qubit hardware, it is necessary to have a solid understanding of microwave systems and components.
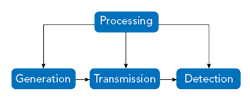
Figure 3 Technical areas related to microwave engineering.
Microwave engineering involves the generation, transmission, processing and detection of microwave signals as shown, generically, in Figure 3. Depending on the application, one or more of the four functional areas described in Figure 3 may be involved. In certain applications, such as microwave heating, detection may not be necessary. On the other hand, detection is essential in fields like radio astronomy. However, in applications like a communication link or a superconducting quantum computer, all four areas play a crucial role. To enhance the understanding of these concepts, they will be expressed in the context of a microwave link in the next section.