
Defence and security authorities around the world are presently pursuing the idea of Network-centric Operations as the key to efficiently identifying and fighting potential threats. For defence applications this is well accepted, but now civil governmental organisations are also using this approach for security applications. The basic idea is to network the available resources and combine the collective capabilities. The enabling elements include: a high performance information grid with access to all appropriate sources; actors/ weapons and other countermeasures with speed of response and high precision; automated command and control (C2) processes enabling high speed decision making and assignment of resources; and integrated sensor grids closely coupled in time to the command and control processes and actors/weapon systems. Figure 1 shows Network-centric Operations building on information grids.

Since information gathering forms the basis and the start of the decision making process, sensors play a vital role in employing the concept of Network-centric warfare. The decisive trends in this field will be considered later in this article, together with the equally important subjects of data processing, communications and electronic warfare. In all phases advanced electronics provides the basis for enabling these capabilities.
Radar Sensors
When it comes to all weather sensing, radar systems are the most important sensors for any platform. The emergence of compact MMIC technology in the ’90s has lead to a revolution of radar systems with regard to capabilities and architecture—the Actively Electronically Scanned Array (AESA) radar. In Europe the AESA radar technology was initially developed and demonstrated in the AMSAR1 program. Operational evidence is proven in high resolution Synthetic Aperture Radar (SAR) such as the multinational SOSTAR,2 with excellent results, and the launch of AESA SAR for space is imminent with the TerraSAR3 satellite. Today, the fourth generation of radar front-end technology is being used in radars such as the CAESAR prototype, a compact multi-function fighter radar.4
Typically, AESA radars are based on a fully modular architecture—the antenna contains several thousands of transmit/receive modules (TRM)—and consists of a number of identical modules, including exciters and processors. All active RF components are solid state and have a much higher intrinsic reliability than conventional HVT (high voltage tubes)-based pulse-Doppler radars. The result is significantly enhanced availability (> factor of 10) due to higher reliability in conjunction with graceful degradation.
Besides the architectural benefit, equally important is that AESA radars offer a new class of operational capabilities such as the highest flexibility in beam scanning and beam forming. GaAs TRMs generate typically 46 dBW of radiated power per square meter of antenna area. In conjunction with very thin antenna arrangements (allowing typically larger antennas), AESA radars have the highest power-aperture product of any radar system, supporting the detection of very small objects at large ranges. Some of the present limitations of GaAs concerning maximum output power and incident damage power will be overcome by the gallium nitride (GaN) components, presently under development.5
Power levels up to 20 W per HPA will become feasible for multi-octave bandwidths up to 20 GHz, while for narrow band X-band the 50 W level becomes realisable. The high energy band gap of GaN will also enable the operation of amplifiers at high supply voltages, reducing some of the present operational constraints. For switches recent results of RF MEMS switch technology have shown very low insertion loss and high isolation with multi-octave performance. This technology may be used for SPDT switches and phase shifters, for example, in SAR and seeker head applications.

Besides the superior equivalent incident radiated power (EIRP) AESA radars offer very large RF bandwidths (> 1 GHz), quasi-instantaneous beamsteering and fully digital beamforming.6 In Homeland Security missions, AESA airborne surveillance radars can provide SAR image resolutions down to < 30 cm in all weather conditions. As an example, Figure 2 shows a high resolution radar image taken with X-band radar.
Another key feature is the potential for aperture sharing and concurrent operation—for example, simultaneous SAR and Moving Target Indication (MTI)—for searching different directions simultaneously. Besides airborne applications, AESA radars are also being used on ships such as the German frigate F 124 APAR,7 for ground applications (MEADS)8 and space borne platforms (TerraSAR and TanDEM-X).
The long-term trend will lead us to radar sensors which can be more highly integrated into airborne structures allowing for wide field-of-view and also to a multi-function sensor which can be shared between radar, communication and EW tasks. Targeted are planar (1D) or conformal (2D) active antenna arrays with very low installation depth and a low number of RF and mechanical interfaces at a small antenna curve radius.

Figure 3 shows a standardized modular transmit/receive module as employed in today’s AESA applications. A key element of this architecture is a very small tile-type transmit/receive module (module size for X-band: 15 × 15 × 5.6 mm) comprising the complete TRM, control electronics and radiating element.9 These antennas will fit perfectly in the outside skin of complex structures such as an aircraft even at positions that could not be utilised before (for example, wings, gear, fin or cabin), while at the same time avoiding increasing the radar cross section. Advanced T/R modules applied to 2D curved structure integrated antennas are shown in Figure 4.
Data Links
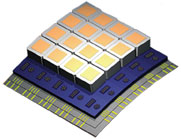
Data links are the vital interconnects between the different elements of the network. Typically, wireless links are used alongside fixed installations and, from the RF-point of view, require similar technologies as described earlier. The upcoming UAV applications will crucially depend on them. The technical trend in this field is also towards total digitalization. This will affect the signal processing part first, but also very quickly the HF-front-end too. The digital-analogue transition is moving close to the antenna front-end10 and this will enable waveforms, protocols and applications which are not currently feasible.
One of the keywords is Software Defined Radio (SDR)11 based on a defined Software Common Architecture (SCA). This approach must be combined with very capable, flexible, digital programmable and very wideband HF hardware. The latter is not yet in place. The spectrum to be covered in the future will need to be from 2 MHz up to the high gigahertz region (more than 60 GHz). This is quite a challenge as the power needed will be over 200 W at low volume and weight.
A very good performing example of today’s state-of-the-art is the MIDS Link 16 power amplifier of the Multi-functional Information Distribution System-Low Volume Terminal (MIDS-LVT), an advanced Link-16 command, control and communication (C3) system.12
A further challenge for data links is to provide secure protected communication and to combine more than one data link. This task has been solved in the Wideband Protected Data Link (WPDL), which is operational in the German Kleinfluggerät ZielOrtung (KZO) drone and in the French System Intermédiaire de Drone MALE (SIDM).13 It combines a wideband link up to 10 MHz and a highly secured command and control data link. Both are uniquely protected by a smart combination of various methods and inherently organize themselves, including the steering of the directed antennas and the GPS-independent localisation of the drone.
With regards to the industrialization and production of data links, three aspects are of particular relevance: limitations due to the International Traffic in Arms Regulations (ITAR), obsolescence of components and the emerging materials. Due to ITAR it will be important for the Europeans to have access to all crucial parts themselves. This is particularly valid as communications move to higher frequencies like Ka-band. Regarding obsolete components there will be a convergence of commercial and military technologies, provided that the emerging market can offer enough and suitable standard components. Finally, modern materials like GaN and SiGe need to be mastered at the series production level.
Assessing Electronic Threats
Significant changes are emerging in the field of electronic warfare. Postulated scenarios during the Cold War assumed a sophisticated threat from radar-guided weapons, both surface-to-air and air-to-air. Today’s asymmetrical warfare scenarios, however, generally assume that an organized, long-range radar-controlled air defence system can quickly be rendered ineffective by a combination of stealth, heavy jamming and physical attack. Once air superiority is achieved, the only air defence assets available to the asymmetrical enemy are those that can be easily concealed and constantly moved around to avoid detection. Typically, such assets will be highly mobile radar-directed SAMs and AAA backed up by larger numbers of EO-guided weapons. Similar scenarios are unfortunately also valid for homeland security and therefore require comparable systems.
In these scenarios, electronic warfare has three major roles:
-
to provide blanket, detailed and real-time battlefield data on the enemy’s actions and intentions, including all kinds of intelligence, especially communications intelligence (COMINT).
-
to completely deny the enemy the full or selective use of the electromagnetic spectrum for target detection, weapon guidance and especially for communication.
-
to provide own forces with a highly reliable self-protection in the event of a successful weapon launch.
In the asymmetrical and especially security related scenario only selective use of these roles may apply, that is, only very selected domains of the EM-spectrum have to be surveyed or to be made unusable.
Electronic Surveillance

The task of electronic surveillance is increasingly being taken over by unmanned aircraft. Thanks to the bandwidth and range of modern data links, the Signal Intelligence (SIGINT) operators can directly control the aircraft receivers in the complete safety of their ground station, no matter how dangerous the mission. An example of such a system is the EADS EHEP sensor shown in a prototype form on the Northrop Grumman GlobalHawk UAV14 in Figure 5. In its operational configuration, this sensor represents an extremely wideband, long-range ELINT/COMINT surveillance asset that can be rapidly deployed anywhere in the world.
Electronic Attack
Electronic jamming of hostile radar sensors is an essential element of any Suppression of Enemy Air Defence (SEAD) strategy. Currently, in the West only the US seems to have significant RF jamming assets in the form of the EA-6B Prowlers, which themselves are nearing the end of their operational life. Because of this, several NATO countries are actively pursuing independent Stand-off Jammer (SOJ) programs based on fighter aircraft, business jets or transport aircraft platforms.
The core of any modern SOJ is a wideband, multi-bit Digital RF Memory (DRFM) capable of following even the most agile radar threat radar. Modern DRFM technology allows multi-gigahertz bandwidth to be achieved with integrated technique generation in small and economical packages. One core element here is the availability of high speed broad bandwidth ADCs. Today 10-bit resolution at 3Gs/s are state-of-the-art.16

Equally important is the transmitter system, which determines the range, number of simultaneous threats and the jamming effectiveness of the SOJ. In the past, vacuum tube technology was the only real option available, but today, solid-state broadband phased arrays based on GaN power sources offer an attractive alternative targeting the > 100 W output level. Figure 6 shows the development of output power versus time for single MMICs, with an inset photo of a 23 W GaN MMIC.
Electro-optical Self-protection: UV and IR Technologies
Today, IR-guided weapons constitute the most serious threat to any aircraft, military as well as civil. Not only are they highly survivable even in an unsymmetrical conflict, but they have also proliferated all over the world and are now in the hands of many terrorist and insurgent forces.
The key to effective IR missile protection is a reliable missile warning system capable of controlling flare dispensing or an IR jamming turret. There are three missile warning technologies: RF detection using pulse-Doppler radar; UV detection of the missile plume; and IR detection of the missile plume. Radar-based warning systems have the advantage of providing time-to-go measurement, but they generally lack the spatial coverage and angular precision of optical warners.

UV missile warners are most widely used due to their low cost, compact construction and relative freedom from false alarms. An example of this type of warner is the MILDS AN/AAR-60 UV warner currently in worldwide operation on many different types of helicopter and fixed-wing aircraft. This detector works by looking for the UV component radiated by the missile plume in the solar-blind region. The main advantage of this approach is that there is practically no natural background interference in this band that can cause false alarms. As an example, Figure 7 shows the MILDS AAR-60 missile warner in the NH90 configuration.
Although UV warners are adequate for most applications, potentially longer ranges can be achieved by detecting the much stronger IR component in the missile signature. In the past, the achievable performance of IR warners was limited by strong IR background clutter caused by the sun, fires, explosions and so on. Extracting the missile signature in this environment requires very high sensor resolution and enormous computing power. Recent advances in both computing power and sensor technology have triggered several IR missile warner programs, perhaps the most advanced of which is the French/German MIRAS multi-colour IR warner for the A400M transport aircraft. These new-generation warning systems have the potential to exploit the range advantages of IR detection while at the same time achieving a very low false alarm rate.
Laser Warning
Laser-guided missiles are of great concern especially to air forces because they are growing in proliferation and virtually immune to known countermeasures. Platform survivability can, however, be greatly increased by warning the crew of laser illumination in time for them to take evasive countermeasures.
Laser warning systems must combine high sensitivity with an extremely large dynamic range. They must also be capable of detecting even single pulses and indicating the threat bearing with a high degree of precision. A particular problem for laser detectors is that of multiple reflections which can falsify the angle measurement of the actual pulse. Many different concepts have been implemented to solve these problems, ranging from diode arrays to delay-line detectors. A newer and more precise measurement method is provided by the Harlid detector,15 which is capable of dual-band detection and high precision angle measurement in a single, compact TO5 housing.
Active IR Countermeasures
Directed IR Countermeasures (DIRCM) represent a highly effective counter to IR-guided missiles. Both lamp-based and laser-based DIRCM systems are available or in development. Compared with flares, these systems are covert, effective against most missile countermeasures and have an essentially unlimited reload capability.
DIRCM systems rely on a missile warning system to perform initial detection and hand off the threat to a jamming turret. This turret then illuminates the missile seeker with a suitably modulated, high power IR beam, which induces an error into the missile tracking system. To be effective against the newest generations of staring missile seekers, a DIRCM must be capable of both multi-spectral jamming and very high power on target, in order to saturate or even physically damage the sensor element. This approach has been extensively investigated in the frame of the French/German FLASH DIRCM program.17
Computers: The Digital Heart
In every case, the managing and controlling element of all the systems that have been mentioned in this article is a computer. Depending on the application, different names are used, such as controller, avionics computer, or signal/data processor. For all ‘mobile applications’ the key requirement besides the computing performance is the power consumption and the heat removal concept. In many applications the limits of available technologies are stressed, requiring sound compromises between hardware capability and software solutions.
With the effort being put into software (SW) development currently easily exceeding that being put into hardware (HW), architectures with a fixed interface (‘API’) between application SW and HW-related SW is becoming standard. This allows users to transfer existing code from one platform to the next, which is especially pertinent with regards to the very short lifetime of Commercial Off The Shelf (COTS) components.
Specific trends today are:
-
the transfer of as much functionality as possible into one computer while demanding a very high reliability, which requires a very low temperature inside the computer.
-
the use of standardized, but very high performance COTS components to comply with the system requirements. Due to cost reasons and the short lifecycles of leading-edge technologies specific computer chip developments are avoided and benefit is taken from the power of other driver markets. Today the game market is dominant with unprecedented performance.
-
increasingly demanding certification rules for safety critical systems, such as aviation or traffic applications. Civil certification standards are entering the military field, for example with the A400M program. Wherever possible, standardized and certified but modular solutions are preferred, leading to families such as Modular Mission Avionic Computers (M2AC).
Very high end performance computers are being developed for the processing of high speed, high volume data, like the radar computer for the Eurofighter (CAPTOR). They are typically realized as array or cluster computers based on ruggedized standard processor chips. Here particularly, the multi-core processor chips of the new game generations will have a strong impact. Environmental impacts like radiation effects or single electron upset (SEU) events are, however, establishing very severe barriers for the use of technologies with line widths below the 120 nm range. New protection or correction mechanisms will be required and are under development.
Conclusion
Gaining information superiority is heavily based on the use of leading-edge technologies. This comprises all areas of electronics: From advanced high frequency materials and circuits to new integrated antenna structures, optical sensors and actors from IR up to UV, digital hardware from simple digitalisation up to highest end ADCs with the continuous need for even more performance, including signal processing and medium to high end computing performance. This, together with the refinement of algorithms and advanced software technologies, has lead to a new generation of networked systems offering unprecedented capabilities. It also means that the effort for research and development as well as for the industrial implementation of these achievements, that is, the ability to manufacture these new systems in a reliable and cost-efficient way, will grow tremendously. As a consequence, increasingly, only companies with a broad technology basis—and the economical power to sustain it consistently—will be able to take the lead in driving technology.
Acknowledgments
Without the contribution of the following colleagues this article would not exist:
H.P. Feldle, C. Hamilton, H. Brugger, A. Bader, L. Belz, U. Pietzschmann, A. Domann, W. Neuhaus and U. Schneider.
References
1. H. Hommel and H.P. Feldle, “Current Status of Airborne Active Phased-array Radar Systems and Future Trends,” Proceedings of the European Radar Conference EuRAD 2004, Amsterdam, The Netherlands, October 11–15, 2004, pp. 121–124.
2. M. Kirscht, “Core Electronics for SOSTAR-X,” Proceedings of the 5th European Conference on Synthetic Aperture Radar, Ulm, Germany, May 25–27, 2004.
3. R. Werninghaus, “The TerraSAR-X Mission,” Proceedings of the 6th European Conference on Synthetic Aperture Radar, Dresden, Germany, May 16–18, 2006.
4. W. Holpp, “The Future of Radar has Begun,” Military Technology, Issue 7, 2006, pp. 100–102.
5. P. Schuh, et al., “20 W GaN HPAs for Next Generation X-band T/R-Modules,” Proceedings of the IEEE IMS, San Francisco, CA, 2006.
6. M. Younis, C. Fischer and W. Wiesbeck, “Digital Beamforming On-receive-only for Radar Applications,” Proceedings of the German Radar Symposium, Bonn, Germany, Sept. 3–5, 2002, pp. 213-217.
7. A.B. Smits and P. v. Genderen, “The APAR Multi-function Radar-system Overview,” Proceedings of IEEE International Symposium on Phased-array Systems and Technology, October 14–17, 2003, pp. 241-246.
8. www.defenseindustrydaily.com/2005/06/34b-development-contract-signed-for-meads/index.php.
9. R. Rieger and H.P. Feldle, “Advanced T/R Module Technology for SAR Applications,” Proceedings of the 6th European Conference on Synthetic Aperture Radar, Dresden, Germany, May 16–18, 2006.
10. I. Aoki, et al., “Fully integrated CMOS Power Amplifier Design Using the Distributed Active-transformer Architecture,” IEEE Journal of Solid-State Circuits, Vol. 37, No. 3, March 2002, pp. 371–383.
11. COMMON STAFF TARGET (CST) for the Tactical Communications-Software Defined Radio; Lt. Col. Zimmermann, 5th Draft, 16 May 2006.
12. I. Aoki, et al., “A Fully Integrated 1.8 V, 2.8 W, 1.9 GHz CMOS Power Amplifier,” Radio Frequency Integrated Circuits (RFIC) Symposium, June 2003, pp. 199–202.
13. “AV Data-links, Tasks, Types, Technologies and Examples,” North Atlantic Treaty Organization (NATO), Research and Technology Organization (RTO), RTO Educational Notes 9 (Development and Operation of UAVs for Military and Civil Applications), April 2000.
14. EuroHawk Nordholz Demonstration: “GlobalHawk Passes German Test,” Flight International, 11 November 2003; “Global Hawk Completes German Tests,” Jane’s Defence Weekly, 12 November 2003.
15. E.G. ALTAS, EADS Product Brochure, “ALTAS 2Q-Advanced Laser Threat Alerting System, 2 Quadrant,” August 2005.
16. EADS Internal Development Using SiGe Technology.
17. EADS Product Brochure, “Flying Laser Self-defence Against Infrared Seekerhead Missiles,” April 2006.
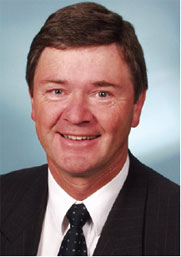
Prof. Dr.-Ing. Heinrich Daembkes
Prof. Dr.-Ing. Heinrich Daembkes studied electrical engineering at the Technical University in Aachen, Germany. After a short period as a young design engineer at Telefunken Radio and TV Systems he took a position at Duisburg University, where he worked on GaAs-based FETs and HEMTs. In 1983 he received his PhD degree on this topic (summa cum laude). Then he started a research career at the AEG Research Centre in Ulm, Germany, working on InP HBT-based optoelectronic receiver ICs, followed by GaAs and SiGe-based technologies and ICs. Until 1996 he was vice president for high frequency electronics at Daimler Research Centre with responsibility for advanced high frequency semiconductor technologies (SiGe, GaAs, InP), optoelectronics, packaging and mm-wave radars. In parallel with this he also became professor at the University of Ulm in 1995. A year later he became a co-founder and CEO of United Monolithic Semiconductors (UMS), a joint venture between Thales and EADS on GaAs-based MMICs, which he turned into a successful commercial enterprise. Since 2003 he has been with EADS Defence Electronics, where he is presently vice president of system and software engineering. He is also active in several organizations including the IEEE and EuMA.