SATELLITE-TERRESTRIAL NETWORKS
A satellite in a 5G satellite access network will have one of two architectures, referred to as bent pipe gNB or regenerative gNB (see Figure 4). The bent pipe simply redirects user data to the ground gNBs using NR protocols, while regenerative satellites have fully functional gNBs. Newer satellites with digital payloads can regenerate an incoming signal, while legacy relay satellites merely shift the incoming signals from the uplink to downlink frequencies, amplifying and transmitting without altering the original content. As shown in the figure, the F1 logical interface in the regenerative satellite represents the connection between a CU and DU in 5G. This helps explain the allocation functions to each of the blocks in the signal chain, where satellites have the potential to provide high bandwidth, low to high latency 5G cellular services to regions across the globe for a variety of use cases.5

Figure 4 Bent pipe (a) and regenerative satellite (b) integration in the 5G access network. Source: 3GPP TR 22.822.
While it is not yet specified by 3GPP, future ground stations may connect multiple satellites from various orbital positions, simultaneously benefiting from the low latency of LEO satellites, the location/positional capabilities of MEO satellites and the coverage and high throughput of GEO satellites. This will require a user terminal able to receive the satellite signal, likely using an active phased array.
HTS ENABLING TECHNOLOGIES
Satellite technology has evolved from the traditional fixed satellite service (FSS) to HTS technologies, which continue to provide more capabilities and services.
Spot Beams and Frequency Reuse
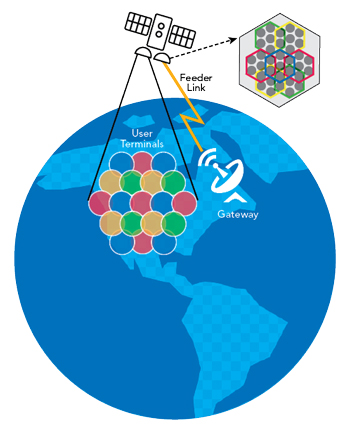
Figure 5 Spot beams and frequency separation improve HTS coverage and capacity.
Where FSS beams are few and extremely large - spanning a continent - HTS satellites yield greater than 20x the throughput within the same frequency allocation, by using multiple spot beams balanced with frequency reuse (see Figure 5). Each spot beam provides more power to a targeted area than the wide beam from an FSS satellite. This enables optimal spectrum usage regardless of the satellite transponder’s operating band (e.g., C-, K- or Ka-Band). To mitigate the risk of interference and signal loss, the spot beams are laid out so neighboring beams are not close in frequency. There is a trade-off between the frequency separation between the spot beams and satellite throughput: the closer in frequency, the more frequency reuse can occur, which gives the satellite more capacity. The concept is like the data rate and capacity increase achieved with mMIMO, where hundreds of active antenna elements and beamforming point multiple beams to users at various locations. However, there is a significant difference between this and spatial diversity: where terrestrial mMIMO systems reduce co-channel interference by increasing the beams, satellites are not in a rich scattering environment, so co-channel interference is a concern. This is mitigated with “four color” frequency reuse (FR4): orthogonality between adjacent beams with disjoint frequencies of different polarizations. The orthogonality is typically preserved to the user terminal.
Multicast
HTS technology is inherently capable of multicast, where one message does not have to be sent individually to a thousand users a thousand different times - rather, just once, providing more efficient use of the spectrum and data resources. Compared to terrestrial wireless services, the coverage area of a satellite beam is large, and the channel codes are long to overcome noise, with the transmitted signal containing information from multiple users. The frame, which may be coded using a DVB-S2X framing protocol, is decoded by a group of users, yielding a multicast transmission.6 As the number of devices receiving the broadcast increases, so do the bandwidth savings. One example of a multicast service is video conferencing, where each participant is a single source multicast to all other participants (i.e., multipoint-to-multipoint). While this is typically a bandwidth hungry process for terrestrial systems, it is relatively straightforward with HTS.
Up the Spectrum
Most recent HTS launches have used Ka-Band transponders. The shift to higher frequencies is to get greater bandwidth, which enables more spot beams. Future generations of satellites will provide terabit per second capacities, which will likely require Q- and V-Band feeder links to be able to aggregate more user traffic and split the coverage into thousands of spot beams.
LEO LOW LATENCY
LEO constellations offer capabilities not viable with single GEO satellites. The major LEO advantages are reduced latency and the increased coverage a LEO constellation provides. A GEO satellite at 35,000 km altitude has an end-to-end propagation delay of 280 ms, a MEO satellite at 10,000 km altitude has a delay of 90 ms and a LEO satellite at 350 to 1,200 km altitude has a delay of 6 to 30 ms. The lower latency of LEO satellites can support a limited range of low latency 5G services; however, the synchronization chain of most low latency 5G services requires much tighter round trip delays and respective timing errors (see Table 1).
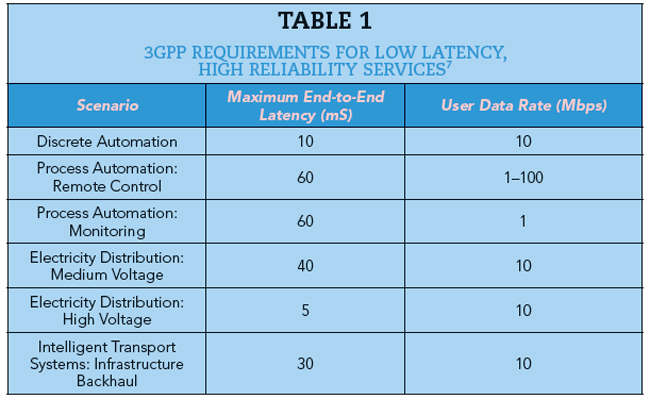
With ubiquitous global coverage, a LEO constellation is best suited to serve mMTC applications. While HTS GEO satellites use a spot beam architecture with frequency reuse to provide targeted service to predesignated areas, a LEO constellation with adequate ground infrastructure can cover the entire globe. While the first LEO constellation, Iridium, went bankrupt shortly after it was launched in 1998, it subsequently provided low data rate services for more than a decade and the constellation was upgraded with a new generation of satellites.8
Several technologies enable a LEO constellation to function, including digital payloads, advanced modulation, frequency reuse, GaN power amplifiers (PA) with high power densities and active phased arrays for beam agility.
LEO Communications
LEO constellations involve “round robin” communications between the ground and satellite, ground stations (G2G), satellites (S2S) and the satellite and ground. These physical links are classified into ground-to-satellite and inter-satellite links. Inter-satellite and ground station communication is another differentiating factor between LEOs and HTS. This net of LEO satellite communication enables tight control of the data transfer among the user and the control and telemetry (e.g., status, diagnostics, configuration).
Instead of maintaining a fixed position in the sky like a GEO, a LEO satellite quickly passes over a ground segment, requiring multiple satellites for consistent coverage of an area. Complex handoffs are required by the ground stations, accomplished with either mechanically-steered reflector antennas or active phased arrays with high gain and directivity. For status updates, beam hopping between a satellite and users to reach remote areas without infrastructure can be supported with G2G links. Tracking of space debris using satellites with cameras and sensors is possible with tight S2S coordination.5
On-Board Processing
Increasing a satellite’s throughput requires adjustments in HTS GEO and LEO satellite architectures. The main change is with the architecture, where a previous bent pipe becomes a regenerative topology. The bent pipe satellite uses transponders with fixed bandwidths that are not finely adjustable, requiring a user to purchase a segment rather than only using what is required for the application. This results in inefficient management of bandwidth and satellite resources.
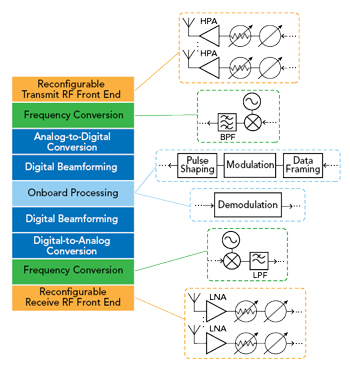
Figure 6 Regenerative satellite onboard signal processing.
Many current satellites use regenerative transponders. Software-defined payloads can be accomplished with on-board processing (OBP) technology or digital transparent processors (DTP). DTPs divide each incoming channel into subchannels of variable width without modifying the form of the received signals. More sophisticated OBP performs beamforming, interference suppression, automatic level control and frequency multiplexing/demultiplexing (see Figure 6). This processing is possible with technologies such as array-fed reflectors or direct radiating arrays, ASICs, high speed serializer/deserializer, digital signal processors and data converters.9 While in orbit, modular and flexible payloads can adjust to changing service needs, configuring to support new applications.
GaN SSPAs
Traveling wave tube amplifiers (TWTA) have historically been used for the PAs in ground stations because of their high output power, necessary to overcome atmospheric propagation losses from the ground to the GEO satellites. A LEO constellation has the benefit of shorter distances, requiring lower transmit power. However, the size constraints in a smaller LEO satellite make the power density of the PA a significant requirement. A solid-state power amplifier, even with relatively low efficiency compared to a TWTA, is often preferred because of its lower launch weight and cost. GaN PAs offer an attractive solid-state option, providing better linearity, lighter weight, a smaller form factor and, in some cases, equivalent efficiency as a TWTA.
Phased Array Antennas
The active phased array is being implemented in space surveillance and tracking systems, where thousands of transmit/receive modules monitor and track space debris. Fitting this technology into smaller ground terminals to track LEO constellations can replace a gimballed antenna with electronic beam steering, changeable scan rates and interference cancellation, and it offers better reliability than a mechanically-steered antenna.
CONCLUSION
Both HTSs and LEO constellations can augment terrestrial 5G networks to ensure seamless connectivity to remote and urban locations, by providing hybrid multiplay and fixed backhaul services. HTS technology is already providing broadband internet around the globe and will likely continue serving this role, while increasing capacity to multi-Tbps with Q- and V-Band feeder links and smaller spot beams. The LEO satellite architecture, while more technically complex, offers a modular high throughput platform with global coverage. Network planners can use the respective advantages of LEO and GEO satellites to supplement terrestrial 5G networks, where needed.
References
- N. Chuberre and C. Michel, “Satellite Components for the 5G System,” 3GPP, January, 2018, Web. https://www.3gpp.org/news-events/1933-sat_ntn.
- “Concept,” SaT5G Project, Web. https://www.sat5g-project.eu/concept/.
- “Communications Infrastructure Upgrade – the Need for Deep Fiber,” Deloitte, July 2017, Web. https://www2.deloitte.com/content/dam/Deloitte/us/Documents/technology-media-telecommunications/us-tmt-5GReady-the-need-for-deep-fiber-pov.pdf.
- “Satellite Reference Use Cases and Scenarios for eMBB,” Deliverable D2.1, SaT5G Consortium, August 2017.
- “Study on Using Satellite Access in 5G,” 3GPP, Technical Report 22.822, V16.0.0, June 2018.
- A. I. Perez-Neira, M. A. Vazquez, M.R. Bhavani Shankar, S. Maleki and S. Chatzinotas, “Signal Processing for High-Throughput Satellites: Challenges in New Interference-Limited Scenarios,” IEEE Signal Processing Magazine, Vol. 36, No. 4, June 2019, pp. 112–131.
- “New Services and Applications with 5G Ultra-Reliable Low Latency Communications,” 5G Americas, November 2018, Web. https://www.5gamericas.org/wp-content/uploads/2019/07/5G_Americas_URLLLC_White_Paper_Final__updateJW.pdf.
- J. Gertner, “The Fall and Rise of Iridium,” The Wall Street Journal, June 2016, Web. https://www.wsj.com/articles/the-fall-and-rise-of-iridium-1464980784.
- P. Angeletti, R. De Gaudenzi and M. Lisi, “From ‘Bent Pipes’ to ‘Software Defined Payloads’: Evolution and Trends of Satellite Communications Systems,” 26th International Communications Satellite Systems Conference, October 2008.