
Figure 6 GH25-10 heterostructure FET (HFET) model validation at 10 GHz on 8 x 125 µm transistor: line = model, circles = measurements.
PDKs for Circuit Simulation
Active device models and passive on-chip components, along with their parametric layout cells (PCells) are organized into PDKs to support MMIC development using these technologies. These PDKs provide simulation-ready device models to design ICs and generate layout masks for fabrication. The PDKs for Microwave Office software are available directly from UMS and include a layout process file (LPF), which defines the material stackup and metallization layers for EM simulation. Designers can adjust the parameters of the active and passive device models, such as gate width/number of fingers or capacitor/inductor values. In addition to a parameterized PCell, models come with a symbol representation for schematic editing. Figure 7 shows components from the GH25 PDK placed in a Microwave Office schematic. A similar PDK will be developed for the GH15 and GH10 process nodes as the finalized models are qualified at UMS.

Figure 7 Various components from UMS GH25 PDK in NI AWR Design Environment software.
Microwave Office software offers a pre-configured example project for FET characterization that designers can use to investigate basic functioning of the transistor model before beginning their design. The default device can be replaced with a nonlinear hot FET model and simulations to observe the device DC and RF performance can immediately be performed. After adding the UMS PDK to the process library, the project layout browser will be populated with the UMS LPF file. The PDK models will appear in the elements browser for user placement in the schematic design window. The FET characterization project is configured to simulate standard device measurements, including DC I/V curves, S-parameters, single- and two-tone swept power such as gain, output power and PAE and power-dependent output load-pull contours (see Figure 8).

Figure 8 Simulation results for a single (8 x 75 µm) 0.25 µm GaN HEMT device, characterized for DC, small- and large-signal RF performance.
With the 0.25 µm PDK installed, the designer is able to apply a combination of linear/nonlinear and load-pull analyses. The recently introduced network synthesis feature in Microwave Office software supports the development of the bias and matching networks from frequency response (S-parameters) or load-pull analysis to achieve optimum power, linearity and/or efficiency performance. This capability was used to determine the appropriate source/load impedance for an 8 x 75 µm (0.25 µm) GaN device operating at 18 GHz, shown in Figure 9. An approximate short circuit was presented to the output of the device at the second harmonic (36 GHz) via a shunt capacitor to improve the peak PAE (~36 percent).

Figure 9 Simulation results for an 18 GHz PA based on a single (8 x 75 µm) 0.25 µm GaN HEMT device.
GaN Technology for 5G Applications

Figure 10 Typical performance of an 8 x 75 µm 0.15 µm transistor at 18 GHz with the output impedance set for optimum Pout and PAE using load-pull measurements.

Figure 11 AM/AM and AM/PM performance of a 8 x 50 µm 0.15 µm transistor at 30 GHz exhibits low phase deviation indicating good linearity.

Figure 12 Example of a 5G 2 W high-power front-end operating from 24 to 31 GHz.
The GH15 technology5 is fabricated on a 4 in. AlGaN/GaN on 70 µm thick SiC substrate wafer. The source-terminated field-plate transistors offer more than 3 W per mm power density at 30 GHz for high-PA (HPA) designs. The process also supports dedicated transistor topologies for cold FET applications such as the switches needed in a front-end module. The typical performance for an 8 x 75 µm GH15 device at 18 GHz is shown in Figure 10. Using load pull to provide the optimum impedance termination for output power and PAE, the transistor exhibits 4 W/mm output power, 13 dB associated power gain (with the Zsource = 50 Ω) and a maximum PAE of nearly 60 percent.
Good transistor linearity is important, especially for telecom applications. A measure of the device’s potential linearity can be obtained through the CW measurements of the transistor’s AM/AM and AM/PM performance as a function of output power. Sweeping the input power driving a 0.15 micron transistor at 30 GHz, which is terminated with a load for maximum power, the device demonstrates low-phase deviation indicating good linearity (see Figure 11).
A 2 W front-end module operating at 24 to 31 GHz covering the 28 GHz 5G band was developed to demonstrate the performance capabilities of the 0.15 micron AlGaN/GaN on SiC technology for mmWave frequencies.6 Two technologies were combined into a plastic package. The module shown in Figure 12 includes the PA and switch realized with 0.15 micron GaN technology and the 0.15 micron GaAs receiver. This device is for telecommunications applications such as high-throughput fixed-access wireless, time-division duplex (TDD) and phased-array antennas.
CW measured power results of the transmit path demonstrate a maximum output power higher than 2 W (33.5 dBm) with 24 percent PAE and 36 dB of insertion gain in the transmit path over the 24 to 31 GHz spectrum. The receiver path provides a noise figure (NF) of 3.6 dB with an associated gain of 20 dB with maximum output power of 30 mW (15.5 dBm). The high-power front-end (HPFE)/Tx linearity has been investigated with several M-quadrature amplitude modulation (M-QAM) signals with 25/50 and 100 MHz channel spacing and using digital predistortion (DPD) leading to 48 dBc adjacent-channel leakage-ratio (ACLR) and 40 dB mean-squared error (MSE) for average output powers ranging from 17 dBm to 25 dBm. The linearity performances have been compared to the ones obtained with two other linear GaAs amplifiers dedicated to point-to-point telecommunications applications: the HPFE presents similar linearity performances associated with a higher efficiency.
An optimized tradeoff in terms of integration, electrical performance and cost was achieved using the mixed-technologies approach, shown in Figure 13. The frequency response of the transmit path shows a gain between 34 to 36 dB over the band, with an output power close to 32 dBm (at 5 dB compression). The PAE varies in the band between 22 to 24 percent (at 5 dB compression). The receive path shows 20 dB gain with a typical NF from 3 to a maximum of 4 dB.
Figure 14 is the large-signal modulated results including ACLR for the transmitter with and without the polynomial DPD. The 256-QAM modulated signal specifications are shown in the lower bottom table, with channel spacing of 56 MHz, RF frequency of 28 GHz, average output power of 23 dBm and peak-to-average power ratio (PAPR) of 9 dB. The top constellation diagram of the Tx output with DPD shows the relevance of a full characterization.
Another example of a GaN Ka-band PA also demonstrates the performance of this process at 29.5 to 36 GHz, in the vicinity of the 5G upper mmWave spectrum (37 to 43.5 GHz) (see Figure 15). The measured performance (CW and 25°C) shows a peak output power greater than 10 W, PAE greater than 25 percent and power gain at Psat greater than 21 dB. The next step at the product level will be to extend the frequency band of this amplifier family to cover the 37 to 40 GHz band for 5G.
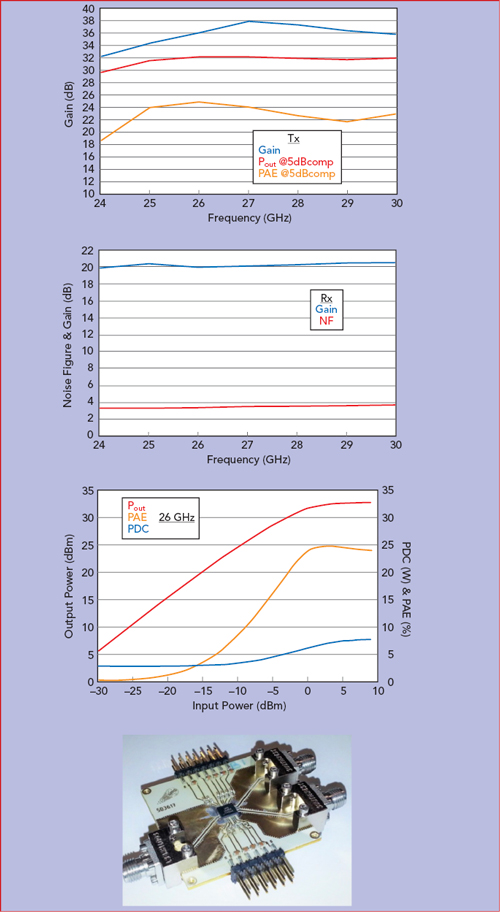
Figure 13 Simulation results for the 5G 2 W HPFE.
Conclusion

Figure 14 Large-signal modulated Tx results showing adjacent channel regrowth with and without DPD.
This article has described emerging GaN on SiC semiconductor technology targeting mmWave frequencies for applications such as 5G. The performance of MMICs designed with this GaN process was demonstrated with two examples: a 10 W Ka-Band (29.5 to 36 GHz) PA and a 2 W integrated front end for 24 to 30 GHz combining a GaN PA with other GaAs functions in a plastic package for 5G applications.
The success of the circuit design was guaranteed by the use of accurate, nonlinear FET models, inclusive of trapping and thermal effects and based on measurements. Load-pull measurements were compared with simulated results to validate model accuracy. The resulting models were organized into a PDK, along with the corresponding layout PCells for use in designing MMICs in a simulator such as Microwave Office software. Along with the UMS PDKs currently available for Microwave Office software, the foundry will fully release the 0.15 micron process in 2019 and is also developing a 0.1 micron GaN process.
References
- O. Jardel, F. De Groote, C. Charbonniaud et al., “A Drain-Lag Model for AlGaN/GaN Power HEMTs,” Proceedings of the International Microwave Symposium, Honolulu, Hawaii, 2007, p. 601.
- A. Benvegnù, “Trapping and Reliability Investigations in GaN-based HEMTs Electronics,” Université de Limoges, 2016.
- S. D. Nsele, L. Escotte, J. G. Tartarin, S. Piotrowicz and S. L. Delage, “Broadband Frequency Dispersion Small-Signal Modeling of the Output Conductance and Transconductance in AlInN/GaN HEMTs,” IEEE Trans. Electron Devices, Vol. 60, No. 4, April 2013, pp. 1372–1378.
- C. Chang et al., “Nonlinear Transistor Modeling for Industrial 0.25 μm AlGaN-GaN HEMTs,” 8th European Microwave Integrated Circuits Conference, October 2013.
- V. Di Giacomo-Brunel et al., “Industrial 0.15 μm AlGaN/GaN on SiC Technology for Applications Up to Ka-Band,“ 13th European Microwave Integrated Circuits Conference, September 2018.
- M. Ayad et al., “Mixed Technologies Packaged High Power Front-End for Broadband 28 GHz 5G Solutions,” 13th European Microwave Integrated Circuits Conference, September 2018.

Figure 15 Output power and PAE over temperature from 25°C to 120°C.