Dual Band Antenna Array for Digital Beamforming in LTE-A and 5G
Beamforming is a phased array technique that enables mobile communications base stations to focus higher power in the direction of user equipment (UE), providing higher signal-to-noise ratio (SNR) and increased data rate. It can also prevent signal degradation from interfering transmitters by nullifying their signals, so mobiles can share the same spectrum with other networks and increase spectral efficiency. This article describes an eight-element linear monopole array designed for and tested at 1.8 and 2.6 GHz. Results meet the goals for steering capability, null and sidelobe suppression and multibeam formation.
The world’s demand for connectivity is rapidly increasing, with mobile subscriptions expected to approach nine billion by the end of 2025, of which some 2.6 billion will be 5G.1 Supporting this fast growing market requires upgrades to operator infrastructure. Beamforming, a well-known technique in defense radar and military communications systems, is being adopted for commercial mobile communications. Beamforming can be thought of as spatial multiplexing. Antenna array elements are weighted in phase and amplitude to modify the radiation pattern as desired. These modifications may be interactive, as in the case of adaptive arrays, or based on predefined switched beams. With beamforming, a base station can direct its antenna gain to communicate efficiently with other devices in the system, while isolating itself from interferers. Spectrum sharing is also possible. Two communication standards operating at the same frequency will not interfere with each other, since each can place beam pattern nulls in the direction of the other.
Global System for Mobile Communications (GSM) was the first attempt to apply beamforming to mobile communications. A base station equipped with an adaptive antenna array processor, allowing full beam uplink and downlink adaptation in every GSM frame, was proposed and tested by Kuchar et al.2 For LTE, beamforming was introduced in 3GPP release 10.3 The standard supports passive arrays with horizontal beamforming and active arrays with 2D and 3D beamforming. Beamforming is becoming more important in the subsequent releases of 3GPP. The 5G new radio (NR) defined in release 15 relies on beamforming and beam management,4 and the 5G NR description considers the possibility of exploiting beamforming in the uplink and downlink transport channels.5 Beamforming requirements are discussed, such as null suppression and steering angle range.6 Digital beamforming enables the efficient evaluation of direction of arrival (DoA) beamforming algorithm performance.
A complete system for testing steering capability, null and sidelobe suppression and multibeam formation using various digital beamforming algorithms is presented in this article. The system is based on commercial transceivers from Analog Devices.7
SYSTEM DESCRIPTION
This system implements beamforming in the digital domain. Calculated complex weights are applied to the received signals at baseband, which requires a complete receiver chain. The system is designed to operate at the LTE-A and 5G NR bands from 1.7 to 1.9 GHz and from 2.5 to 2.7 GHz.8 5G NR includes sub-6 GHz bands, referred to as FR1 and mmWave bands, designated FR2.9
The number of array elements determines the directivity of the main beam and its half-power beamwidth. As the number of elements increases, the directivity increases and half-power beamwidth decreases.10 This test bed comprises an eight-element antenna array, an RF front-end and a field-programmable gate array (FPGA) digital processor (see Figure 1). The antenna array is composed of wideband monopoles linearly distributed with uniform inter-element spacing. RF signals transmitted by the UE are received by the antenna array. These signals have different phases and amplitudes, depending on the position of the UE relative to the base station. Dynamically weighting the received signals produces maxima and nulls in the radiation pattern, determined by the weights. This system can evaluate and test the performance of horizontal beamforming algorithms, as well as the performance of new algorithms. To do this, eight full receiver chains are used, with the eight I/Q pair outputs digitized for post processing. The beamforming weights are calculated and applied to the digital baseband signals in the FPGA. Summation of the weighted signals provides the best SNR at the angle of the main beam and the worst SNR at the angle of the null in the radiation pattern.

Figure 1 Beamforming test bed.
MONOPOLE ANTENNA ARRAY
CST Microwave Studio 2017 was used for the design and simulation of the antenna array.11 The single element is a monopole with a rectangular shape and rounded vertices of radius Rcor1. The monopole is printed on Rogers RO4003C 0.06 in. thick, which has a relative dielectric constant of 3.38 and loss tangent of 0.0027 (see Figure 2). It is fed from an edge-mounted SMA connector through a 50 Ω microstrip line. The transition between the feed line and the rectangular antenna element is elliptical, with a minor axis length Lgap. The width of the element and its length are Wmono and Lsub, respectively. The antenna is backed by a leaf-like ground plane of width Wgnd and length Lgnd. The dimensions used in the design are Wmono = 57 mm, Lmono = 36.5 mm, Rcor1 = 9 mm, Lgap = 6.5 mm, Wgnd = 57 mm and Lgnd = 24 mm. The ground surface is modified with 10 corrugations in the bottom edge to minimize coupling to the coax. The antenna has a metallic reflector 44 mm from the substrate to direct the antenna’s power in the boresight direction. Confirming the monopole’s performance, Figure 3a shows the simulated and measured impedance matching from 1.7 to 2.7 GHz, and Figure 3b shows the simulated and measured H-plane radiation patterns at 2.6 GHz.

Figure 2 Top (a) and bottom (b) of the single element antenna.

Figure 3 Simulated vs. measured performance of the single element antenna: |S11| vs. frequency (a) and 2.6 GHz E-plane pattern (b).

Figure 4 Eight-element monopole array.
For horizontal beamforming, the single monopole is extended to a linearly distributed eight-element array with uniform element spacing (see Figure 4). Because the antenna is wideband, the choice of inter-element spacing was challenging. The separation was set to 83 mm, which is a half-wavelength at 1.8 GHz. The eight elements are uniformly fed using a Mini-Circuits ZB8PD-362-S+ eight-way power divider. Figure 5 shows the simulated and measured radiation patterns at 1.8 and 2.6 GHz with each element uniformly excited.
RF FRONT-END
Because beamforming is performed digitally, a complete receiver chain is needed. The Analog Devices AD9361 wideband receiver was selected, as it meets most common communication standards, including LTE-A, contains two separate receive chains and operates from 70 MHz to 6 GHz.12 As the demonstration system requires eight receiver channels to capture the signals from the eight antenna elements, the full receiver uses two Analog Devices’ FMCOMMS5 transceiver boards with dual FMC connectors, compatible with the Zynq ZC702 FPGA board from Xilinx (see Figure 1). Each transceiver board has four dedicated receiver channels driven by a single local oscillator (LO), requiring two transceiver boards for the eight receive channels.

Figure 5 Monopole array H-plane patterns at 1.8 (a) and 2.6 (b) GHz, simulated vs. measured.
To evaluate digital beamforming in the uplink, weights are applied to the received signals. The signal coming from the antenna element passes through a coaxial cable into an internal low noise amplifier. The amplified signal is down-converted by a mixer, and the I/Q signal pair is digitized by a 12-bit, third-order, continuous time delta-sigma modulator analog-to-digital converter (ADC). After digital down-conversion and filtering with a decimation finite impulse response filter, the signal is ready for post processing.
In digital beamforming, the relative phase between elements is critical. If the eight receivers are not synchronized in phase, accurate post processing beamforming is not possible. There are two challenges: the first, to ensure that all LOs are at the same frequency; the second, to ensure all are synchronized in phase. The LO driving both transceivers in each AD9361 chip is an Analog Devices ADF5355BCPZ phase-locked loop (PLL) with integrated VCO. A 40 MHz temperature compensated crystal oscillator (TCXO) is used as the PLL reference clock.
If the four PLLs were driven from the same reference clock and configured with the same N-divider, all the PLLs would oscillate at the same frequency. Each of the two transceivers on the same FMCOMMS5 board are driven by the same PLL. To synchronize the two transceiver boards, the TCXO reference clock on one, the master, is buffered with an ADCLK846B fanout buffer and passed via a coaxial cable to the external reference input of the other board, the slave (see Figure 6). This locks the two PLLs at the same frequency. However, while the RF paths for the eight channels are almost identical, there is still a length difference between the paths, in addition to the path difference between the master and slave boards.

Figure 6 Master/slave setup (a) for synchronizing the phase between the two FMCOMMS5 boards (b).
The phases of all eight elements must be aligned before processing signals from the antenna. To do so, the eight elements’ receivers are connected to a ZB8PD-362-S+ eight-way splitter. The input of the splitter is connected to the transmitter channel of one of the transceivers. After coarse phase synchronization, a manual fine alignment of the receiver channel phases is performed on each board, adding a fixed angle to each channel as required to align them. Knowing that the two LOs are at the same frequency, the four signals of the master board now lead or lag the four channels of the slave board by a constant phase, which is also evaluated and compensated manually. After this alignment, the power divider is removed and the antenna array is connected to the eight receivers using equal length cables. For applications where more channels are needed, in the case of massive MIMO, a clock distribution network can be used to synchronize the frequency and phase among all the boards.
TESTING

Figure 7 Monopole array and transceiver boards in the anechoic chamber.
To test the full system and validate the outcomes, real signals at various angles are recorded, beamforming weights are calculated on the fly and radiation patterns after post processing are recorded. Measurements are conducted in the controlled environment of an anechoic chamber using a Vivaldi transmit antenna, fed from the transmitter channel in the FMCOMMS5 master board (see Figure 7). The transmitter needs nine seconds to transmit one burst of data, so the motor that rotates the Vivaldi antenna is adjusted to an angular speed of 1/9 degree per second as it rotates from -90 to +90 degrees. The processing system captures data from the eight elements simultaneously at each step, accumulating data from the eight signals at 181 angles. The master and slave FMCOMMS5 boards are mounted on the opposite side of the receive antenna array, as shown in Figure 7. Before starting the measurements, phase calibration is performed as described.
The test mimics a UE signal transmitted toward a base station antenna array. Baseband data in LTE-A and 5G NR are modulated with quadrature amplitude modulation (QAM),13,14 so the test signal is quadrature phase shift keying (QPSK) modulated, with a length around 2,400 samples. At each stop angle, a burst of modulated QPSK data is sent through the Vivaldi antenna and received through the eight-element array. The receiver’s ADC sampling rate is set to 30 MSPS, with the channel bandwidth at 5 MHz. After recording data, beamforming weights are applied to the stored signals. In this test, the weights are calculated based on the linearly constrained minimum variance (LCMV) beamformer. An LCMV beamformer passes signals from preferred directions with maximum gain while blocking interference from other directions. The total output power after applying the LCMV weights is minimized under the constraints of source and interference directions.15 The steering capability of the array is limited to between -60 and +60 degrees for a total azimuth view angle of 120 degrees.
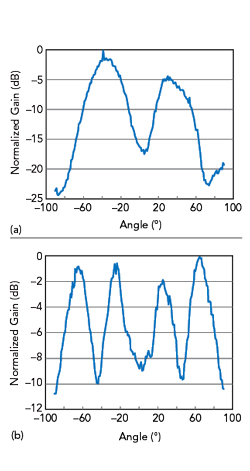
Figure 8 Normalized radiation patterns at 1.8 (a) and 2.6 (b) GHz, derived from the baseband signal after applying LCMV beamforming weights.
Several scenarios were tested, with two shown here: 1) The normalized radiation pattern with the source at 35 degrees and interferers at 10 and 65 degrees, operating at a carrier frequency of 1.8 GHz (see Figure 8a); 2) The normalized pattern at 2.6 GHz with two sources at 60 and 35 degrees and an interferer at 0 degrees (see Figure 8b).
CONCLUSION
An eight-element antenna array test bed for digital beamforming in LTE-A and 5G was developed and evaluated. The system, using commercial wideband transceivers covering the FR1 LTE-A and 5G NR bands, was tested in an anechoic chamber with an LCMV beamformer at 1.8 and at 2.6 GHz. The measurements agree with the predicted performance. This work will continue, using the system to evaluate DoA estimation algorithms for LTE-A and 5G base stations in noncontrolled environments.
ACKNOWLEDGMENT
This work is a collaboration between the Lebanese University and Universitat Politècnica de València and partially supported by the Lebanese University. It has been supported by a scholarship from Erasmus Mundus Welcome Program and by the Spanish Ministry of Economy and Competitiveness, under the project TEC2016-78028-C3-3-P.
References
- Ericsson, Ericsson Mobility Report, November 2019, www.ericsson.com/4acd7e/assets/local/mobility-report/documents/2019/emr-november-2019.pdf.
- A. Kuchar, M. Taferner, M. Tangemann and C. Hoek, “Field Trial With a GSM / DCS1800 Smart Antenna Base Station,” IEEE VTS 50th Vehicular Technology Conference, September 1999.
- “Overview of 3GPP Release 10,” Vol. 0.2.1, 3GPP, June 2014.
- “Services Provided by the Physical Layer, 3GPP TS 38.201 V15.0.0 Release 15,” 3GPP, July 2018.
- “3GPP TS 38.300 V15.2.0 Technical Specification 3rd Generation Partnership Project; NR and NG-RAN Overall Description,” 3GPP, June 2018.
- ”R4-1700199_NR Specific Beamforming New Requirements for NR,” 3GPP, January 2017.
- P. Zhou, “Target Networks in 5G Era Embracing Mobile Network 2020s,” Huawei, 2017.
- “TS 136 104 V10.2.0 Technical Specification LTE; Evolved Universal Terrestrial Radio Access (E-UTRA); Base Station (BS) Radio Transmission and Reception,” 3GPP, May 2011.
- “TS 38.101-1 V15.0.0 Technical Specification 3rd Generation Partnership Project; NR; User Equipment (UE) Radio Transmission and Reception,” 3GPP, July 2018.
- C. A. Balanis, “Antenna Theory: Analysis and Design,” Wiley, 2015.
- “CST Studio Suite 2017,” Dassault Systemes.
- “AD9361 Reference Manual,” Analog Devices.
- “TS 36.211 version 10.0.0 Release 10; Evolved Universal Terrestrial Radio Access (E-UTRA); Physical Channels and Modulation,” 3GPP, January 2011, p. 9.
- “3GPP TS 38.211 V15.2.0 Technical Specification 3rd Generation Partnership Project; NR; Physical Channels and Modulation (Release 15),” 3GPP, July 2018.
- B. D. Van Veen and K. M. Buckley, “Beamforming: a Versatile Approach to Spatial Filtering,” IEEE ASSP Magazine, Vol. 5, No. 2, April 1988, pp. 4–24.